U.S. patent application number 16/496403 was filed with the patent office on 2021-04-15 for lidar measuring device.
The applicant listed for this patent is METEK Meteorologische Messtechnik GmbH. Invention is credited to Christoph Bollig, Ernst Brinkmeyer, Gerhard Peters.
Application Number | 20210109218 16/496403 |
Document ID | / |
Family ID | 1000005342550 |
Filed Date | 2021-04-15 |
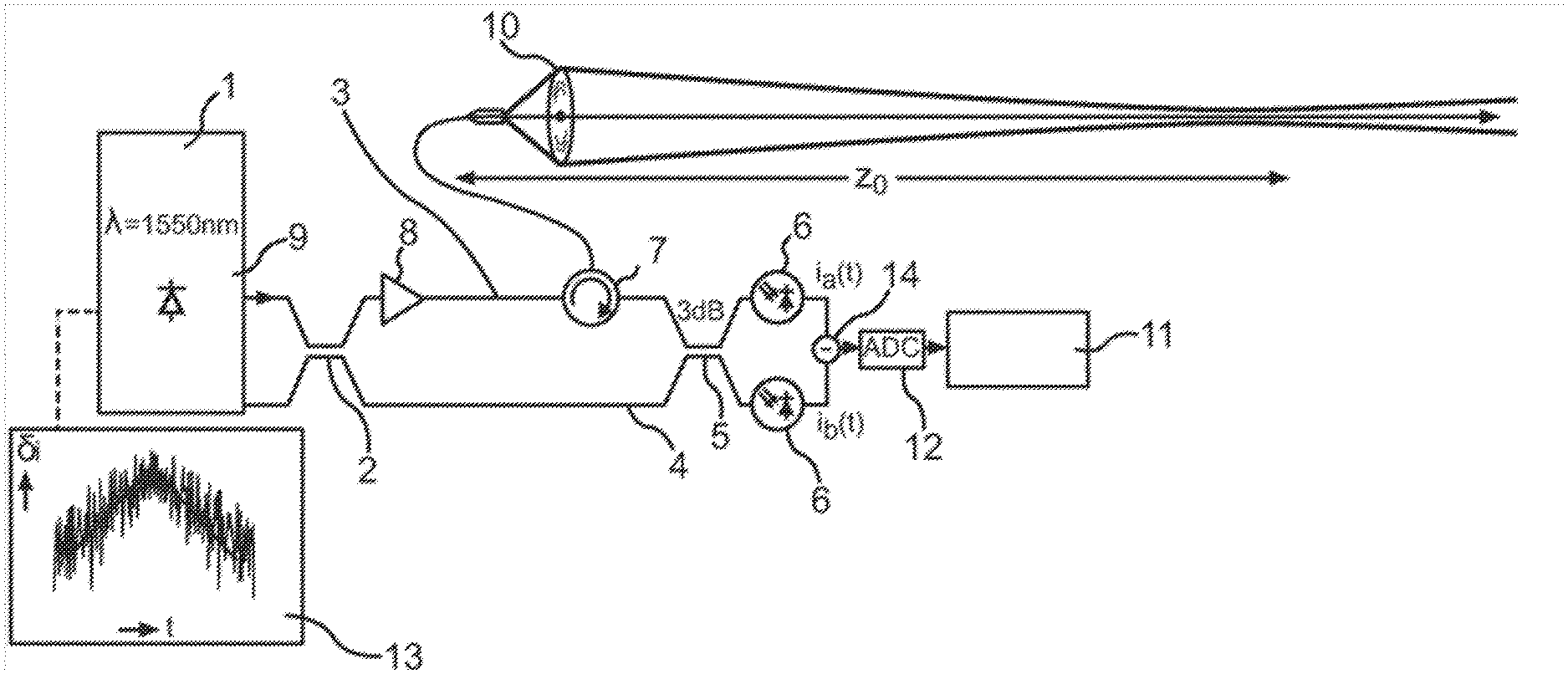
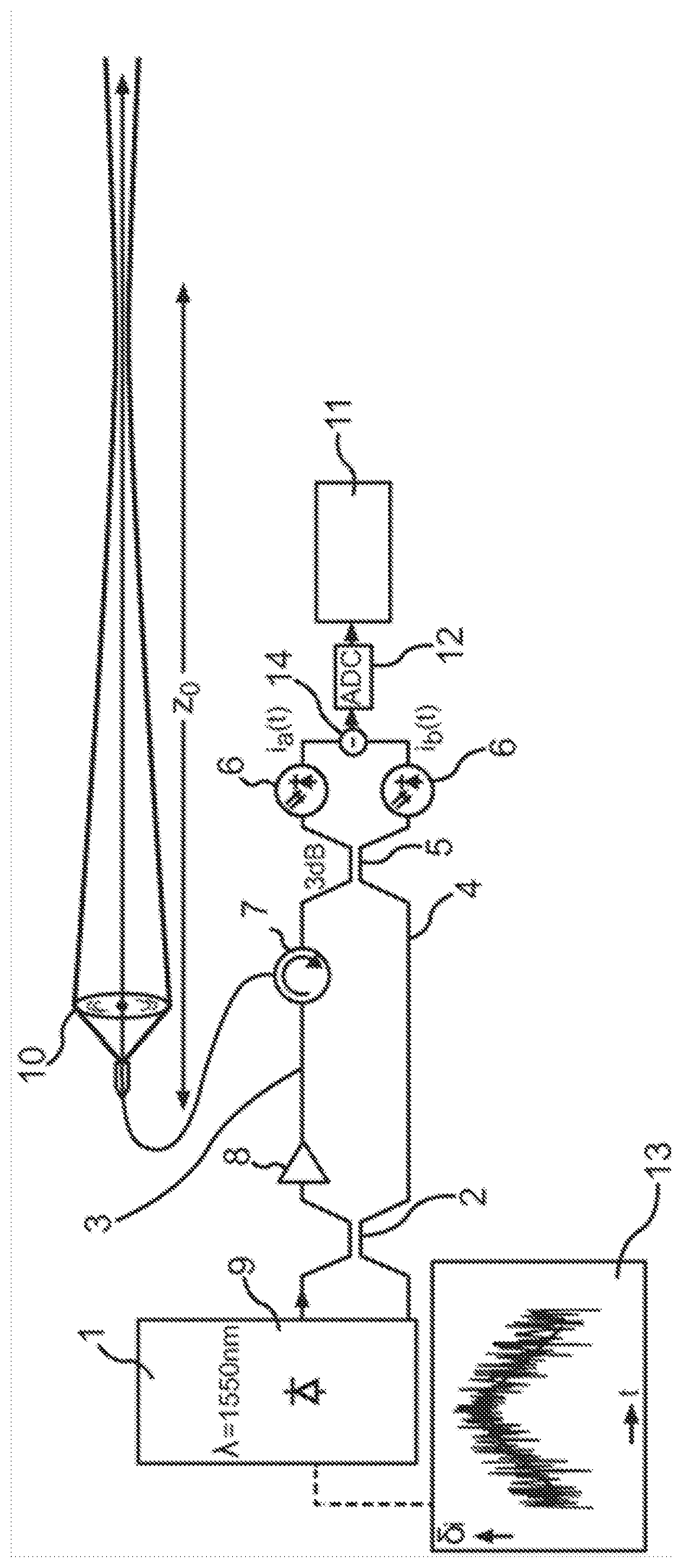









View All Diagrams
United States Patent
Application |
20210109218 |
Kind Code |
A1 |
Peters; Gerhard ; et
al. |
April 15, 2021 |
LIDAR measuring device
Abstract
A LIDAR measuring device and a method for determining the speed
of particles in a measuring volume includes a narrowband continuous
wave laser light source (1), which emits light which is coupled
into a measuring branch (3) and a reference branch (4). The light
coupled into the measuring branch (3) is at least partially emitted
by a transmitting device in the direction of the measuring volume
such that the emitted light is at least partially scattered and/or
reflected by the particles in the measuring volume. A part of the
scattered and/or reflected light is then received by a receiver
device and is coherently superimposed with the light leaving the
reference branch (4), and the resulting light beam is directed onto
a detector (6) to generate a detector signal characteristic for the
resulting light beam. Finally, the speed of the particles in the
measuring volume is determined in an evaluation unit (11) by taking
into account the detector signal.
Inventors: |
Peters; Gerhard; (Hamburg,
DE) ; Brinkmeyer; Ernst; (Buchholz, DE) ;
Bollig; Christoph; (Gottingen, DE) |
|
Applicant: |
Name |
City |
State |
Country |
Type |
METEK Meteorologische Messtechnik GmbH |
Elmshom |
|
DE |
|
|
Family ID: |
1000005342550 |
Appl. No.: |
16/496403 |
Filed: |
March 20, 2018 |
PCT Filed: |
March 20, 2018 |
PCT NO: |
PCT/EP2018/057050 |
371 Date: |
September 20, 2019 |
Current U.S.
Class: |
1/1 |
Current CPC
Class: |
G01S 7/4911 20130101;
G01S 7/4814 20130101; G01S 7/484 20130101; G01S 17/88 20130101;
G01S 17/58 20130101 |
International
Class: |
G01S 17/58 20060101
G01S017/58; G01S 7/481 20060101 G01S007/481; G01S 7/4911 20060101
G01S007/4911; G01S 7/484 20060101 G01S007/484; G01S 17/88 20060101
G01S017/88 |
Foreign Application Data
Date |
Code |
Application Number |
Mar 22, 2017 |
DE |
10 2017 106 226.2 |
Claims
1-16: (canceled)
17. A LIDAR measuring device for determining the speed of particles
in a measuring volume, comprising: a narrowband continuous wave
laser light source (1) that emits light that is coupled into a
measuring branch (3) and a reference branch (4); a transmitting
device, wherein the light coupled into the measuring branch (3) is
at least partially emitted by the transmitting device in a
direction of the measuring volume in such a way that the emitted
light is at least partially scattered and/or reflected by the
particles in the measuring volume; a receiver device, wherein at
least a part of the scattered and/or reflected light is received by
the receiver device and is coherently superimposed with the light
leaving the reference branch (4) to form a resulting light beam; a
detector (6) to receive the resulting light beam and to generate a
detector signal characteristic for the resulting light beam; an
evaluation unit (11) to determine a speed of the particles in the
measuring volume by taking into account the detector signal; a
control device (13) to vary the frequency of the light emitted by
the laser light source (1), wherein the evaluation unit (11) is
configured to determine the speed of the particles in at least one
measuring region of the measuring volume on the basis of a spectral
analysis of the detector signal by taking into account a predefined
frequency modulation.
18. The LIDAR measuring device according to claim 17, wherein the
control device (13) changes a current strength of the supply
current for the laser light source (1) in order to vary the
frequency of the emitted light.
19. The LIDAR measuring device according to claim 17, wherein the
laser light source (1) has a laser diode (9), wherein an injection
current of the laser diode (9) is modified in order to vary the
frequency of the emitted light.
20. The LIDAR measuring device according to claim 17, wherein the
frequency modulation is selected in such a way that the laser light
source (1) emits light with a coherence length of from 0.1 to 100
m.
21. The LIDAR measuring device according to claim 17, wherein the
laser light source (1) emits light with a coherence length of from
1 to 50 m.
22. The LIDAR measuring device according to claim 17, the control
device (13) of the laser light source impresses an additional
signal in such a way that an additional time-linear portion is
added to the frequency of the emitted light.
23. The LIDAR measuring device according to claim 22, wherein the
time-linear change in the frequency of the emitted light is
selected to be large enough that the sign of the difference does
not change despite a Doppler shift of the frequency of the received
light as compared to the transmitting frequency, said shift being
caused by movement of the particles.
24. The LIDAR measuring device according to claim 17, wherein the
control device (13) changes the current strength such that the
frequency of the light emitted by the laser light source (1) is
varied linearly upward and downward.
25. The LIDAR measuring device according to claim 17, wherein the
control device (13) is configured such that the emitted light is
pulsed, wherein pulse lengths are selected such that they are
longer than what corresponds to the desired spatial resolution,
such that the spatial resolution continues to be determined by the
frequency modulation of the laser light source.
26. The LIDAR measuring device according to claim 17, wherein an
optical switch is arranged in the measuring branch (3).
27. The LIDAR measuring device according to claim 26, wherein the
optical switch is an acoustico-optical modulator.
28. The LIDAR measuring device according to claim 17, wherein at
least one element polarizing the light is arranged in the reference
branch (4).
29. A use of a LIDAR measuring device according to claim 17 to
record a wind speed and/or a wind direction on the windward side of
a wind turbine.
30. A use of a LIDAR measuring device according to claim 17 to
record a wind speed and/or a wind direction on the windward side of
a wind turbine, wherein the measuring device is attached to a
spinner of the wind turbine.
31. A method for determining the speed of particles in a measuring
volume, comprising: transmitting light with a narrowband continuous
wave laser light source (1) and coupling the transmitted light into
a measuring branch (3) and a reference branch (4); at least
partially emitting the light coupled into the measuring branch (3)
by a transmitting device in the direction of the measuring volume
in such a way that the emitted light is at least partially
scattered and/or reflected by the particles in the measuring
volume; receiving by a receiver device at least a part of the
scattered and/or reflected light and coherently superimposing the
at least a part of the scattered and/or reflected light with the
light leaving the reference branch (4) to form a resulting light
beam; directing the resulting light beam onto a detector (6) to
generate a detector signal characteristic for the resulting light
beam; determining a speed of the particles in the measuring volume
in an evaluation unit (11) by taking into account the detector
signal, wherein the frequency of the light emitted by the laser
light source (1) is varied with a control device (13) and a
spectral analysis of the detector signal is carried out in the
evaluation unit (11) in order to calculate the speed of the
particles in at least one measuring region of the measuring volume,
by taking into account a predefined frequency modulation.
32. The method according to claim 31, wherein the variation of the
frequency of the light emitted by the laser light source (1) takes
place based on a control signal generated by the control device
(13), said control signal corresponding to a pseudo-noise signal
caused by a predefined frequency function.
33. The method according to claim 31, wherein the variation of the
frequency of the light emitted by the laser light source (1) takes
place based on a control signal generated by the control device
(13), said control signal containing a time-linear portion.
Description
[0001] The invention relates to a LIDAR measuring device as well as
a method for determining the speed of particles in a measuring
volume. With the help of the described device as well as the
method, the measurement of a wind speed in a remote measuring
volume is realized with a narrowband continuous wave laser light
source. The light emitted by the continuous wave laser light source
is coupled into a measuring branch and a reference branch, wherein
at least a part of the light coupled into the measuring branch is
at least partially emitted by a transmitting device in the
direction of the measuring volume in such a way that the emitted
light is at least partially scattered and/or reflected by the
particles in the measuring volume such that at least one part of
the scattered and/or reflected light is received by a receiver
device. This scattered and/or reflected received light is then
coherently superimposed with the light leaving the reference
branch, and the resulting light beam is directed onto a detector to
generate a detector signal characteristic for the resulting light
beam. The speed of the particles in the measuring volume is
determined in an evaluation unit by taking into account the
detector signal.
[0002] In general, land-based measuring methods for determining the
wind speed and the wind direction for different altitude ranges of
the atmosphere, for example to monitor industrial pollutant
sources, for weather forecasts, as well as for atmospheric and
climate research have been known for many years. In this context,
for example, so-called SODAR measuring systems are used for wind
measurements, wherein they use the Doppler effect with soundwaves
which are scattered back from the atmosphere. Due to the use of
wind energy, which is increasingly gaining in importance in recent
years, a new significant application area has opened up for such
measuring methods to measure wind speed and direction in areas
remote from the actual measuring device. In addition to the known
SODAR systems, so-called LIDAR measuring systems in which light is
used instead of sound and which are partially superimposed on the
SODAR have been used for such measurements recently.
[0003] LIDAR (light detection and ranging) is a method, related to
radar, for the optical measurement of distance and speed as well as
the measurement of atmospheric parameters. LIDAR systems for the
atmospheric measurement emit a laser beam and detect the light
scattered back from the atmosphere. The distance away from the
location of the scattering is calculated from the light travel time
of the signals. In particular, clouds and dust particles in the air
scatter the laser light and enable a high-resolution selection and
distance measurement of clouds and aerosol layers.
[0004] Furthermore, it is possible to determine the wind speed
prevailing at a location remote from the measuring device with the
LIDAR measuring systems via the measurement of light which is
scattered and/or reflected on particles moved by wind. This type of
measurement can be used, in particular, to measure the prevailing
wind speed upstream of a wind turbine up to a few hundred meters
away and to adapt control parameters of the wind turbine based on
the measured wind speed. On one hand, the most effective use of a
wind turbine possible and, on the other hand, system overloads due
to special wind events should hereby be assured. In this manner,
emergency measures can be introduced in a timely manner, for
example with extreme wind gusts, in order to extensively prevent
damage to the wind turbine.
[0005] The known LIDAR measuring systems for determining wind
speeds are based on the detection and evaluation of the Doppler
frequency shift of the scattered light effected by the scattering
of laser light on the particles moved with the wind in comparison
to emitted light (cf.: C. Weitkamp, LIDAR-range-resolved optical
remote sensing of the atmosphere, Springer 2005).
[0006] Typically, the Doppler frequency shift is determined
interferometrically by means of superimposition of the scattered
light received by the measuring device with light which is coupled
into the measuring device by the laser light source by means of a
second optical path. To this end, highly coherent laser light
sources which emit light with coherence lengths of several hundred
meters, in which the bandwidth of the frequency is in the sub-MHz
range, are used with the known LIDAR measuring devices.
[0007] In order to ensure the required and/or desired spatial
resolution, essentially two different procedures are known. A first
technical variant provides for the use of continuous wave laser
sources which are focused on to the respectably desired target area
with the help of a suitable optical system, for example a lens or a
telescope. A corresponding technical solution is described in WO
2005/1142531. In the event that the desired focus area should be
changed, this is achieved with the help of mechanical elements
which usually have, however, a comparatively complex design and are
expensive. Moreover, such adjusting elements carry the risk of
additional faults, primarily in long-term operation.
[0008] A second technical variant for achieving and changing the
desired spatial resolution is based on the use of pulsed laser
sources. In this case, the location determination takes place by
means of a travel-time measurement of the laser pulses. In this
manner, multiple sequential measuring regions can be measured by
the measuring system with a pulse, the pulse width of which
determines the spatial resolution which is, for example, 30 m for
pulses with a width of 200 ns. In this case, the individual
sequentially measured measuring regions can each represent
subsections of the entire measuring volume to be tested.
[0009] However, the illumination of an individual measuring region
by a pulse, said illumination only occurring for a brief time,
results in poor signal-to-noise ratios. Furthermore, the frequency
resolution and thus also the resolution of the wind speed is
limited due to the short pulse widths.
[0010] Starting from the previously described measuring systems, WO
2014/072514 discloses a further technical solution for recording
the wind speed in a remote spatial area, which achieves a change of
the spatial resolution with alternative technical measures. It is
essential for this technical solution that a narrowband light
source is used, which is modulated with a defined phase pattern,
instead of the laser light sources typically used. Firstly, a
relatively low transmitting power is required due to the use of a
continuous wave laser source as compared to pulsed LIDAR. In
addition, the spatial resolution does not have to be balanced
against the speed resolution with a pulse LIDAR, but rather both
parameters can be selected independently of one another within wide
limits. In comparison to focused continuous wave LIDAR (CW-LIDAR),
this solution furthermore offers the advantage that the spatial
resolution does not become coarser as the distance increases but
instead is independent of distance.
[0011] An essential component of the described LIDAR measuring
device is a modulator, which is based, for example, on the
electro-optical effect in a suitable material and which ensures
desired phase modulation of the frequency of the light emitted by a
laser diode. This modulator represents a comparatively expensive
component, which furthermore requires a suitable actuation as well
as supply of energy, in relation to the measuring device.
[0012] A problem with the previously known LIDAR measuring systems
is that a spatial resolution can only be assured either with
comparatively extensive complexity and components somewhat
susceptible to faults, or comparatively expensive opto-electric
components are required. This makes the provision of reliable and
simultaneously economical LIDAR measuring devices significantly
more difficult, particularly for the use of wind energy.
Furthermore, it is often not possible with the known LIDAR
measuring devices to differentiate, in a satisfactory manner, a
positive Doppler shift caused by different movements of the
particles in the measuring region from a negative Doppler shift.
Further measuring devices must often be used as a supplement to the
LIDAR measuring device.
[0013] With consideration of the technical solutions known from the
prior art for recording speed as well as the direction of movement
of particles in a remote measuring volume with the help of a LIDAR
measuring system and the previously explained problems, the object
of the invention is to further develop a LIDAR measuring system
such that a highly precise speed measurement of particles moved in
the remote measuring region is possible with the help of a
comparatively simple construction. The technical solution to be
indicated in this case should primarily enable a comparatively
simple adjustment and setting of the spatial resolution. It should
further be assured that a direction of movement of the particles in
the measuring region can also be determined reliably with simple
means, particularly without the additional measuring devices and/or
complex components being absolutely necessary.
[0014] The aforementioned object is achieved with a device
according to claim 1 as well as a method according to claim 13.
Especially suitable uses of the invention are indicated in claims
11 and 12. Advantageous embodiments of the invention are the
subject matter of the dependent claims and will be explained in
greater detail in the following description with occasional
reference to the exemplary embodiments.
[0015] The invention is based on a LIDAR measuring device for
determining the speed of particles in a measuring volume with a
narrowband continuous wave laser light source, which emits light
modulated in its optical frequency and then coupled into a
measuring branch and a reference branch. The light coupled into the
measuring branch is at least partially emitted in the direction of
the measuring volume by a transmitting device in such a way that
the emitted light is at least partially scattered and/or reflected
by the particles in the measuring volume such that at least one
part of the scattered and/or reflected light is received by a
receiver device. Furthermore, the received light is coherently
superimposed with the light leaving the reference branch, and the
resulting light beam is directed onto a detector to generate a
detector signal characteristic for the resulting light beam. The
speed of the particles in the individual measuring regions of the
measuring volume is then determined in an evaluation unit by taking
into account the detector signal. The invention is characterized in
that the frequency of the light emitted by the laser light source
is varied with a control device in a predefined manner, and a
spectral analysis of the detector signal is carried out in the
evaluation unit in order to calculate the speed of the particles in
the individual measuring regions of the measuring volume, while
taking into account the predefined frequency change and/or
frequency modulation. The expressions "frequency modulation" and/or
"frequency change" should be understood as having the same meaning
with reference to the description of the invention.
[0016] When evaluating the received beam, a Doppler frequency shift
is determined and evaluated between the beam entering the
respective measuring region and the received, scattered and/or
reflected beam. In contrast with the known technical solutions, it
is essential for the invention that the frequency of the light
emitted by the laser light source be changed and/or modulated. Due
to the modulation of the frequency, it is possible, with the help
of a known function, to select the spatial resolution and to
precisely define the distance between the measuring device and the
respective measuring region to be measured with the particles
contained therein.
[0017] This is achieved in that a spectral broadening and
deformation of the at least extensively monochromatic light is
achieved based on the change in the frequency of the light emitted,
wherein the bandwidth determines the coherence length. Thus, it is
possible to implement various coherence lengths and thus spatial
resolutions with the same laser light source. A narrowband laser
light source, which is preferably light with a bandwidth which is
less than 1 MHz, preferably less than 100 kHz, especially
preferably less than 10 kHz, is used as the laser light source. The
measuring device preferably contains an optical amplifier, which
can be located behind the laser source before the split-off into
the measuring and reference branch or in the measuring branch.
[0018] In an advantageous manner, the power supply of the laser
light source is modulated directly, which ultimately leads to a
variation of the frequency of the emitted light beam and thus of
the power spectral density. Due to the technical solution used,
that is the frequency modulation of the emitted light, a highly
precise measurement of the speed of the particles moved in a
remotely placed measuring volume can be implemented without the use
of comparatively expensive components.
[0019] According to a special embodiment of the invention, the
laser light source is designed as a laser diode, which emits at
least approximately monochromatic light. In a preferred manner, the
injection current of the laser diode is varied in this case with
the help of a suitably designed control device and/or with the help
of the control signal generated by the control device. This
variation of the power supply leads, on one hand, to a typical,
insignificant change in the output power and, on the other hand, to
a desired variation in the optical frequency and/or wavelength of
the emitted light. In this manner, it is possible, for example, to
achieve a change in the optical frequency per change in the
injection current change in a range of from 0.5 to 1 GHz/mA with a
DFB laser, which emits light in a wavelength range of from 1530 to
1570 nm, preferably of 1550 nm. However, such lasers typically have
a line width of from 1 to 3 MHz, which is not especially
well-suited for the described application. If smaller line widths,
for example of 50-100 kHz, are required, so-called extended cavity
lasers (ECLs) can be used in an advantageous manner, which have a
tuning coefficient which is greater than 0.1 GHz/mA.
[0020] Instead of implementing the intended frequency modulation by
means of a modulation of the injection current, special laser
diodes with frequency tuning ranges and special tuning electrodes
can be used.
[0021] In a special further embodiment, the evaluation unit is
implemented such that, on one hand, the known frequency function
and, on the other hand, an additional time shift value are
considered in evaluating the generated detector signal, in addition
to the frequency modulation of the light emitted by the laser light
source. The time shift value is selected such that the result of
the function corresponds to the detector signal, which would result
with selection of a reference branch, which has a travel time
modified by the time shift value and thus also a correspondingly
changed optical path length, as compared to the actual reference
branch.
[0022] This design is based on the knowledge that the detector
signal only contains the information required for the evaluation
regarding particles in the measuring range corresponding to the
actual optical path length of the reference branch and the
coherence length, which only represents a part of the entire
illuminated region, but also respective information regarding
particles and other illuminated regions, which do not correspond to
the selected measuring region and/or the selected spatial
resolution in the measurement currently being implemented.
[0023] In an advantageous manner, a continuous wave laser light
source is used, the emitted light of which has a coherence length
in a range of from 0.1 to 100 m based on the frequency modulation.
Especially suitable is a coherence length in a range of from 1 to
100 m and very especially suitable in a range of from 1 to 50 m.
When selecting the laser light source and/or defining the coherence
length, it should be considered that coherent interference on the
detector is only possible for the scattered light of particles
and/or only the reflected light from objects, for which the optical
path length of the measuring branch within the coherence length
matches the optical path length of the reference branch. For this
reason, a high spatial resolution, which is adjustable through the
selection of the coherence length, is achieved by means of a
greatly limited coherence length. In contrast, the scattered light
reaching the detector array, said scattered light coming from
particles located outside the coherence range, leads only to a
broadband background in the detector signal, which can lead to a
small reduction in the signal-to-noise ratio, but does not impact
the spatial resolution. If the coherence range is less than the
maximum expansion of the measuring volume, only a partial section,
namely of the respective measuring region, is measured, and a
particular spatial resolution can be achieved by means of its
optical length, the coherence length, by means of shifting of the
coherence range, for example with the help of an optionally
available device to change the optical path length of the reference
branch, which is preferably done with computing means. In an
advantageous manner, the spatial resolution is independent of the
distance of the respectively tested measuring region.
[0024] According to a further special embodiment of the invention,
the control device is designed such that the variation of the
frequency of the light emitted by the laser light source takes
place based on a control signal generated by the control device,
said control signal corresponding to a pseudo-noise signal caused
by a predefined frequency function. In this manner, it is possible
to modulate the frequency of the emitted light in a desired manner
and, in doing so, to particularly adapt the optical power spectral
density in a desired manner. Because the frequency function used in
the control device is known, the respective laser light source can
be used to implement various coherence lengths and thus various
spatial resolutions.
[0025] In a special further embodiment of the invention, the
frequency of the light emitted by the continuous wave light source
is varied such that it has a time-linear portion, the latter being
generated, for example, by an approximately time-linear portion in
the laser diode injection current. This can be combined with the
previously described pseudo-noise profile.
[0026] This measure enables, in a preferred manner, sign detection
upon the frequency shift between the frequency of the emitted and
received light caused by the Doppler effect.
[0027] The signal derived from the scattered and/or reflected light
received does not contain the information on the frequency of the
light but instead on the difference as compared to the frequency of
a reference light, which was routed from the laser light source via
the reference branch. The speed of the particles moved in the
measuring volume is derived from this difference with consideration
of the Doppler effect. In the simplest case, the reference light is
branched off of the transmitter and has the same frequency as the
emitted light.
[0028] In this context however, it must be considered that the
frequency difference may have a positive or negative sign depending
on the direction of movement of the particles in the measuring
volume toward the LIDAR or away from the LIDAR. Due to the
modulation, provided according to the invention, of the frequency
of the light emitted by the laser light source, it is conceivable,
in a preferred manner, to superimpose the frequency of the light
with a further linear time-dependent signal such that the
transmitting frequency of the light being emitted toward the
measuring volume changes linearly. In an advantageous manner, it is
provided in this case that a difference between the transmitting
frequency and a frequency of the scattered and/or reflected light
received by the receiver device is selected to be large enough that
a sign of the difference does not change despite a Doppler shift of
the frequency of the received light as compared to the transmitting
frequency, said shift being caused by the movement of the
particles. As soon as the difference between the transmitting
frequency and the receiving frequency is selected to be
particularly large in a suitable manner, the Doppler shift can no
longer change the sign of the difference and the uniqueness is
assured in relation to the direction of movement of the particles
in the measuring volume.
[0029] With the previously described design of the linear frequency
modulation, the frequency resolution can be worsened in an
undesirable manner. This can be prevented by means of a
corresponding limit on the slope of the linear frequency
modulation. The potentially alternating sign of the difference
between the transmitting frequency and the receiving frequency can
be detected by means of a triangular frequency modulation and
separate analysis of the ascending and the descending branch. The
total appears in one branch and the difference between the Doppler
shift and the frequency shift, based on travel time, appears in the
other branch.
[0030] In a further particular embodiment of the invention, the
frequency of the light emitted by the continuous wave laser light
source is changed and/or modulated upward and downward. The
resulting frequency shift of the received signal as compared to the
transmitting signal then depends on the distance between the
measuring region and/or the volume region, in which the scattering
takes place, and on the Doppler shift. The frequency resolution is
determined, as in the previously described method, with
superimposed stochastic and linear frequency modulation by means of
the slope of the modulation and the spatial resolution. The spatial
resolution in this case is generated in a known manner by means of
focusing, particularly with the help of suitably adjustable optical
elements. Due to the triangular modulation of the transmitting
signal, the sign of the frequency shift may also be determined in a
preferred manner during the evaluation of the difference
frequencies in the ascending and descending branch, as previously
described. In comparison with the known methods which provide the
determination of the wind direction with an additional measuring
device, particularly with a weather vane, or the determination of
the distance portion based on a separate measurement, the
triangular modulation in this case represents an especially
economical method for achieving a uniqueness when determining the
direction of movement of the particles in the measuring volume. A
further advantage of the triangular modulation is that not only the
Doppler shift but also the precise effective distance of the focus
can be derived by means of evaluation of the different frequencies
in the ascending and descending branch. Depending on whether the
Doppler shift causes a signed change of the difference frequencies
or not, the Doppler shift is equal to half the difference (total)
of the difference frequencies and the distance of the focus is
equal to a fourth of the light speed multiplied by the total
(difference) of the difference frequencies. It is easy to decide
which case it is, because the approximate distance of the focus is
known.
[0031] A special further embodiment of the invention is
characterized in that an optical switch, for example an
acousto-optic modulator, is used in the measuring branch. The pulse
lengths in this case should be selected such that they are longer
than what corresponds to the desired spatial resolution such that
the spatial resolution continues to be determined by the frequency
modulation of the laser source. With this operating mode, the
detector signal is no longer continuously available. Despite of
this, there are advantages in certain application cases, for
example when inner reflexes from the measurement setup cannot be
sufficiently suppressed, when a strongly incoherent background
greatly worsens the signal-to-noise ratio due to strong scattering
outside of the measuring volume, or when special properties of the
optical amplifier in pulse mode allow for the anticipation of a
significant advantage. The advantages of a measuring system
designed according to the invention with a pulsed laser exist in
that longer distances can be achieved as compared to an un-pulsed
operation, without limiting normal pulsed systems with respect to
spatial resolution and speed resolution.
[0032] A special further embodiment of the invention is
characterized in that the light emitted by the laser light source
is routed to an optical amplifier, particularly an erbium-doped
fiber amplifier (EDFA), before the branching of the measuring and
reference branch. Furthermore, polarization-maintaining fibers are
preferably used for the measuring device designed according to the
invention. If such fibers are not used, it is generally conceivable
to provide at least one light-polarizing element, preferably a
polarization regulator, particularly in the reference branch.
[0033] A LIDAR measuring device designed according to the
invention, as has previously been described, is especially suitable
for recording wind speed and/or a wind direction prevailing on the
windward side of a wind turbine. In this context, it is further
conceivable that a corresponding LIDAR measuring device is mounted
on the spinner of a wind turbine and thus the speed and wind
direction of the wind flowing to the wind turbine can be detected
at a sufficient distance to enable, on one hand, an effective
operation of the wind turbine and, on the other hand, to prevent
damage to the wind turbine, for example due to sudden wind gusts,
primarily by adjusting the pitch of the rotor blades in order to
reduce the load or by initiating an emergency switch-off.
[0034] Furthermore, the invention also relates to a method for
determining the speed of particles in a measuring volume, with
which light is transmitted with a narrowband continuous wave laser
light source and coupled into a measuring branch and a reference
branch. The light coupled into the measuring branch is at least
partially emitted by a transmitting device in the direction of the
measuring volume in such a way that the emitted light is at least
partially scattered and/or reflected by the particles in the
measuring volume. At least a part of the scattered and/or reflected
light is then received by a receiver device of the LIDAR measuring
device and is coherently superimposed with the light leaving the
reference branch, and the resulting light beam is directed onto a
detector to generate a detector signal characteristic for the
resulting light beam. The speed of the particles in the measuring
volume is finally determined in an evaluation unit by taking into
account the detector signal. The method according to the invention
is characterized in that a frequency of the light emitted by the
laser light source is varied, in a predefined manner, with a
control device, and a spectral analysis of the detector signal is
carried out in the evaluation unit in order to calculate the speed
of the particles in the individual measuring regions of the
measuring volume, while taking into account the predefined
frequency modulation. In an advantageous manner, a laser diode, the
injection current of which is varied based on a suitable control
signal, is used as the laser light source.
[0035] Based on a corresponding variation of the frequency of the
light emitted by the laser diode, it is possible to change the
spatial resolution of the LIDAR measuring device without having to
use a different laser light source or performing a mechanical
adjustment.
[0036] In a special embodiment of the method according to the
invention, it is further provided that the variation of the
frequency of the light emitted by the laser light source takes
place based on a control signal generated by the control device,
said control signal corresponding to a noise signal precisely
caused by a predefined frequency function.
[0037] In the following, the invention is explained in greater
detail by means of exemplary embodiments with reference to the
figures, without limiting the general concept of the invention. The
following is shown:
[0038] FIG. 1: principle structure of a LIDAR measuring device
designed according to the invention;
[0039] FIG. 2: curve of the electrical power spectral density as a
function of the frequency;
[0040] FIG. 3: curve of a modulated frequency over a section of
time;
[0041] FIG. 4: curve of the modulated injection current over a
section of time.
[0042] FIG. 1 schematically shows a LIDAR measuring device designed
according to the invention. The LIDAR measuring device has a
continuous wave laser light source 1 with an output power of 10 mW,
which emits light with a wavelength of 1550 nm. The light emitted
by the laser light source 1 is coupled, on one hand, into an at
least partially fiber-optically defined measuring branch 3 and, on
the other hand, into a completely fiber-optically defined reference
branch 4 with the help of a fiber-optic coupler, which takes on the
function of a beam splitter 2.
[0043] The optic fibers used are preferably single mode fibers with
low damping and a field radius of advantageous 5 .mu.m.
Polarization-maintaining fibers, so-called PM fibers, are
preferably used.
[0044] A further fiber-optic coupler 5 is arranged at the end of
the measuring branch 3 and the reference branch 4 for combining the
light from the measuring branch 3 and from the reference branch 4.
The light leaving the reference branch 4 is coherently superimposed
with the received light and the resulting light beam is routed to
the detector 6. According to this exemplary embodiment, the
detector has two separate photodetectors, which are preferably
designed as InGaAs detectors. Characteristic signals are generated
for the detected beam with the help of the detector 6, wherein the
two signals are subtracted from one another in a subtraction
element 14 in order to eliminate faults. The difference signal is
then supplied to an evaluation unit 11, in which the different
signal is evaluated to detect the presence and/or movement of
particles, by means of an analog-digital converter 12.
[0045] If no PM fibers are being used, an adjustable, fiber-optic
polarization regulator and a diverting path, for example in the
form of a wound section of the fiber, are provided in the reference
branch 4.
[0046] The measuring branch has an erbium-doped fiber amplifier
with a preferred output power of 1 W and an optic circulator 7,
arranged sequentially, as the amplifier 8.
[0047] From the circulator 7, the light coupled into the measuring
branch 3 is routed to a transmitting and receiving lens 10, with a
focal length of 250 mm for example, and from there focused in the
direction of the measuring volume to be measured and/or the
respective measuring region.
[0048] The beam scattered and/or reflected in particles in the
measuring volume then reaches, at least to a certain extent, the
transmitting and receiving lens 10 of the LIDAR measuring device.
In the exemplary embodiment shown here, the transmitting and
receiver device are combined in the transmitting and receiving lens
10. The scattered and/or reflected light hereby received is
subsequently coupled into the remaining fiber-bonded section of the
measuring branch 3 via the circulator 7.
[0049] The continuous wave laser light source 1 has a laser diode 9
and is coupled to a control device 13, which varies the injection
current of the laser diode 9 in a suitable manner such that the
frequency of the light emitted from the laser diode is also
changed. A frequency function is stored in the control device 13 as
a function of the desired spatial resolution such that the light
emitted by the laser light source 1 is changed, particularly
broadened and deformed, as compared to the light typically emitted
by the laser diode 9 by means of the modulation as a function of
the frequency function in the optical power spectral density. With
the help of the control device 13, the frequency of the emitted
light is thus changed based on a suitable frequency function
defining a pseudo-noise signal. Due to the consideration of various
time-shifting values during the evaluation by the evaluation unit,
the detection of particles and the movement thereof can be carried
out separately in a spatially resolved manner, in purely
mathematical terms, for various measuring regions remote from the
lens 10 at different distances, without changing the described
setup.
[0050] Essential for the invention is that the frequency of the
light emitted by a laser light source 1 is precisely varied such
that no phase modulator is required for the laser light source and
furthermore no acoustico-optical modulator (AOM) is required, in
contrast to the technical solutions known from the prior art.
[0051] In order to achieve a suitable frequency modulation such
that the phase modulator used according to the prior art can be
dispensed with and a modulation of the frequency of the emitted
light is still achieved, which achieves the same effect as with the
phase modulation .THETA.(t) used with known measuring systems, the
current frequency of the laser diode according to
f mom ( t ) = 1 2 .pi. d .THETA. ( t ) dt ##EQU00001##
[0052] must be changed, wherein .THETA.(t) corresponds to the
respective phase. To do this, a modulation of the injection current
of
.delta. i mod ( t ) = 1 .gamma. tun f mom ( t ) = 1 2 .pi. .gamma.
tun d .THETA. ( t ) dt ##EQU00002##
[0053] is necessary.
[0054] However, this leads to an undesired modulation of the laser
output power
.delta. P 0 , mod ( t ) = .beta. .delta. i mod ( t ) = .beta. 2
.pi..gamma. tun . d .THETA. ( t ) dt ##EQU00003##
[0055] wherein .beta.=dP.sub.0/di characterizes the slope
efficiency, for which 0.2 mW/mA can be assumed as a reference
value.
[0056] From the laser output power P.sub.0+.delta.P.sub.0,mod, a
part .rho. is coupled into the LO branch, wherein the
modulation-based variation of the transmitting power is
insignificant:
P L O + .delta. P L O ( t ) = .rho. ( P 0 + .delta. P 0 , m o d ( t
) ) = .rho. P 0 + .rho..beta. .delta. i m o d ( t ) = .rho. P 0 +
.rho. .beta. 2 .pi. .gamma. tun d .THETA. ( t ) dt ##EQU00004##
[0057] With an ideal, balanced reception, the variation of the LO
power is not noticeable. With a real, balanced reception, an AC
signal of
.delta. i Det = .delta. P LO ( t ) = P LO .beta. 2 .pi..gamma. tun
P 0 d .THETA. ( t ) d t ##EQU00005##
[0058] with the photodiode response sensitivity R=1 A/W is
received. As a comparison, a detector current results for a
(corrected) useful signal of power P.sub.signal with the root mean
square
i signal , eff = 1 2 P signal P LO ##EQU00006##
[0059] For a rough comparison, the root mean square of
f.sub.mom(t), where
f.sub.mom.sup.2(t)=1/2f.sub.max.sup.2
[0060] is estimated. This results in
( .delta. i Det ) 2 _ ( i signal , eff ) 2 _ .apprxeq. ( P L o
.beta. f max .gamma. tun P 0 ) 2 1 2 P signal P L O = ( .beta. f
max .gamma. tun P 0 ) 2 P LO P signal ##EQU00007##
[0061] Where
[0062] .epsilon.=0.01; .beta.=0.2 mW/mA; .gamma..sub.tun=100
MHz/mA; P.sub.0=20 mW; f.sub.max=10 MHz; P.sub.LO=100 .mu.W, this
results in
( .delta. i Det ) 2 _ ( i signal , eff ) 2 _ .apprxeq. 1 0 - 14 W P
signal ##EQU00008##
[0063] This comparison makes it clear that the modulation-based
noise background does not need to be considered with such a root
mean square. In addition, it should be considered that the signal
is narrowband, for example 100 kHz, while the modulation-based
noise is broadband and is concentrated on the low-frequency
frequency domain, as is shown in FIG. 2.
[0064] With the phase modulation known in the prior art with the
electro-optical phase modulator, the predefined phase .THETA.(t) is
used for correction. The phase modulator is actuated with a signal
proportional to the phase. This can result in a scaling error such
that the phase impressed on the optical signal is {tilde over
(.THETA.)}(t)=.eta..sub.scale.THETA.(t). In this context, it is
known that values of .eta..sub.scale=0.9 to 1.1 are
non-critical.
[0065] With the direct modulation, provided according to the
invention, of the laser light source with the help of a suitable
frequency function, a modulation signal according to
.delta. i m o d ( t ) = 1 .gamma. tun f mom ( t ) = 1 2 .pi.
.gamma. tun d .THETA. ( t ) d t ##EQU00009##
[0066] is impressed on the injection current. If a scaling error
occurs in this case as well, to which the following applies
.delta. i ~ mod ( t ) = .eta. scale 1 2 .pi..gamma. tun d .THETA. (
t ) d t = 1 2 .pi. .gamma. tun . d ( .eta. scale .THETA. ( t ) ) d
t ##EQU00010##
[0067] the effects are thus the same. This directly means that the
LIDAR measuring device according to the invention, with which a
frequency modulation is carried out directly on the laser light
source, is implementable such that the previously used phase
modulator can be omitted.
[0068] As shown already with the previous statements, it is known
from the prior art to subject the optical wave emitted by a laser
light source to a predefined phase modulation .THETA.(t) with the
help of a phase modulator and to shift the optical frequency in an
interferometer arm by f.sub.AOM by means of an acoustico-optical
modulator (AOM). A fixed target at a distance z then provides a
detector signal
u ( t ) .varies. exp { j [ 2 .pi. f AOM t + .THETA. ( t - 2 z c ) -
.THETA. ( r ) ] } ( 1 ) ##EQU00011##
[0069] If the target moves at speed v in the +z direction, a
Doppler shift
f Doppler = - 2 .lamda. v ##EQU00012##
also occurs:
u ( t ) .varies. exp { j [ 2 .pi. f AOM t + 2 .pi. f Doppler t +
.THETA. ( t - 2 z c ) - .THETA. ( t ) ] } ( 2 ) ##EQU00013##
[0070] For correction, this signal can be multiplied by the known
function
h ( t ; z ) .varies. exp { - j [ .THETA. ( t - 2 z c ) - .THETA. {
t ) ] } ( 3 ) ##EQU00014##
[0071] The corrected signal for position z is then purely
sinusoidal:
u.sub.cnt(t)=u(t)h(t;z).varies.exp{j.left
brkt-bot.2.pi.f.sub.AOMt+2.pi.f.sub.Dopplert.right brkt-bot.}
(4)
[0072] In the spectrum, it provides a narrow line for
f.sub.AOM+f.sub.Doppler.
[0073] If this correction function h(t;z) is used, the target is at
locations
z 1 < z - .DELTA. z LC 2 or z 2 > z + .DELTA. z LC 2 ,
##EQU00015##
thus the electrical power spectral density is broad. The width
depends on .THETA.(t). Likewise, the variable .DELTA.z.sub.LC
depends on the phase pattern .THETA.(t) and is characterized as the
spatial resolution, constrained by the modulation-based low
coherence. Corresponding phase patterns, e.g. for .DELTA.z.sub.LC=5
m, 10 m, 15 m, can be found numerically by means of a special
iteration process. Targets within range
[ z - .DELTA. z LC 2 , z + .DELTA. z LC 2 ] ( 5 ) ##EQU00016##
[0074] thus provide approximately a narrow spectrum for the
frequency f.sub.AOM+f.sub.Doppler having a width
.delta. f = q T , ##EQU00017##
which is essentially provided by the measuring time T. Outside of
this range, a broad spectrum is obtained.
[0075] According to a further embodiment of the invention, the
frequency of the light emitted by the laser light source,
particularly a laser diode, is changed into two types. In this
context, there is a stochastic modulation of the frequency and, in
addition, the frequency of the light emitted by the laser light
source is changed in a time-linear manner. The following
applies:
f.sub.laser=f.sub.laser,0+.GAMMA.t (6)
[0076] Thus a detector signal is obtained according to
u ( t ) .varies. exp { j [ 2 .pi. .GAMMA. 2 z c t + 2 .pi. f
Doppler t + .THETA. ( t - 2 z c ) - .alpha. ( t ) ] } ( 7 )
##EQU00018##
[0077] In contrast to equation (2), the location-dependent,
chirp-based frequency
f chirp ( z ) = .GAMMA. 2 z c ##EQU00019##
then occurs here in place of the constant frequency f.sub.AOM.
[0078] If there is a correction where h(t;z) according to eq. (3),
a result according to eq. (4) is obtained:
u ent ( t ) = u ( t ) h ( t ; z ) .varies. exp { j [ 2 .pi. .GAMMA.
2 z c t + 2 .pi. f Doppler t ] } ( 8 ) ##EQU00020##
[0079] Because the chirp rate .GAMMA. and the location z are
defined and thus are known, the Doppler frequency f.sub.Doppler can
be determined therefrom.
[0080] If the location of the target is then changed, but the
correction function h(t;z) is retained, modified chirp-based
frequencies as follows are thus obtained at the edges
z .+-. .DELTA. z LC 2 ##EQU00021##
of the spatial resolution
f chirp ( z .+-. .DELTA. z LC 2 ) = .GAMMA. 2 z c .+-. .GAMMA.
.DELTA. z LC c = f chirp ( z ) .+-. .delta. f chirp 2 ( 9 )
##EQU00022##
[0081] Within the interval
[ z - .DELTA. z LC 2 , z + .DELTA. z LC 2 ] , ##EQU00023##
the frequency in the spectrum is thus smeared by
.delta.f.sub.chirp. To ensure that the Doppler resolution is not
substantially worsened as compared to the solution known from the
prior art which uses an acoustico-optical modulator (AOM), the
following must apply
.delta.f.sub.chirp.ltoreq..delta.f (10)
[0082] This results in
.GAMMA. 2 .DELTA. z LC c .ltoreq. .delta. f .GAMMA. .ltoreq. c 2
.DELTA. z LC .delta. f ##EQU00024##
[0083] If the largest-possible
.GAMMA. = c 2 .DELTA. z LC .delta. f ##EQU00025##
.GAMMA. is selected and measurements where .GAMMA.=|.GAMMA.| and
.GAMMA.=-|.GAMMA..sym. are carried out, which correspond to a
triangular frequency modulation, spectral lines after correction
are obtained with the frequencies
f + = .GAMMA. 2 z c - f Doppler = z .DELTA. z LC .delta. f - f
Doppl and f = - .GAMMA. 2 z c - f Doppler = z .DELTA. z LC .delta.
f - f L ( 12 ) ##EQU00026##
[0084] For the Doppler frequency, the following thus applies:
f Doppler = - f 1 2 - f 2 4 z .DELTA. z LC .delta. f ( 13 )
##EQU00027##
[0085] If one of the frequencies is f.sub..+-.<.delta.f and thus
not determinable, it again applies that f.sub.Doppler can also be
determined from the other one:
If f - > f + , then f Doppler > 0 f Doppler = f - - z .DELTA.
z LC .delta. f ( 14 a ) If f - < f + , then f Doppler < 0 f
Doppler = z .DELTA. z LC .delta. f - f + ( 14 b ) ##EQU00028##
[0086] A few specific calculation examples are shown in the
following:
.DELTA. z LC = 10 m , .delta. f = 0.5 MHz , q = 2 T = 4 .mu.s ,
.GAMMA. = 7.5 10 12 Hz / s = 7.5 MHz / .mu.s , .GAMMA. T = 30 MHz ,
.DELTA. i tot = .GAMMA. T / .gamma. tun = 37.5 .mu.A ( i ) z = 10 m
, f Doppler = 10 MHz f 1 = 9.5 MHz , f = 10.5 MHz ( ii ) z = 50 m ,
f Doppler = 10 MHz f - = 7.5 MHz , f - = 12.5 MHz ( iii ) z = 100 m
, f Doppler = 10 MHz f - = 5 MHz , f - = 15 MHz ( iv ) z = 20 m , f
Doppler = 1 MHz f 1 = 0 MHz , f - = 2 MHz ( 15 ) ##EQU00029##
[0087] For the frequency modulation of the current supply of the
laser light source, particularly of the injection current of a
laser diode, the following considerations are significant. In this
manner, a suitable frequency modulation and the linear frequency
chirp can be implemented.
[0088] With a predefined phase pattern .THETA.(t) in order to
implement a spatial resolution .DELTA.z.sub.LC and a desired linear
frequency chirp (chirp rate .GAMMA.), the total phase modulation of
the optical wave must be
.THETA..sub.tot(t)=.THETA.(t)+1/22.pi..GAMMA.t.sup.2
[0089] and the corresponding frequency modulation and current
modulation
f tot ( t ) = 1 2 .pi. d .THETA. tot ( t ) dt = 1 2 .pi. d .THETA.
( t ) dt + .GAMMA. t ##EQU00030## .delta. i mod ( t ) = 1 .gamma.
tun f tot ( t ) = 1 2 .pi. .gamma. tun d .THETA. ( t ) dt + .GAMMA.
.gamma. tun t ##EQU00030.2##
[0090] FIGS. 3 and 4 each show exemplary measurements, which prove
the previous calculations. FIG. 3 in this case shows the curve of
the frequency f.sub.tot in MHz and FIG. 4 shows the modulated
injection current .delta.i.sub.mod in .mu.A, each over a timeframe
of 4 .mu.s (.GAMMA.=7.5 MHz/.mu.s, .gamma..sub.tun=800 MHz/mA)
LIST OF REFERENCE NUMERALS
[0091] 1 Laser light source
[0092] 2 Beam splitter
[0093] 3 Measuring branch
[0094] 4 Reference branch
[0095] 5 Fiber-optic coupler
[0096] 6 Detector
[0097] 7 Circulator
[0098] 8 Amplifier
[0099] 9 Laser diode
[0100] 10 Lens
[0101] 11 Evaluation unit
[0102] 12 Analog-digital converter
[0103] 13 Control device
[0104] 14 Subtraction element
* * * * *