U.S. patent application number 16/967851 was filed with the patent office on 2021-02-18 for rational design of redox mediator for fast and energy-efficient charging of sulfur cathodes.
The applicant listed for this patent is The Board of Trustees of the Leland Stanford Junior University. Invention is credited to Zhenan Bao, Yi Cui, Min Ah Lee, Yuchi Tsao.
Application Number | 20210050624 16/967851 |
Document ID | / |
Family ID | 1000005208460 |
Filed Date | 2021-02-18 |



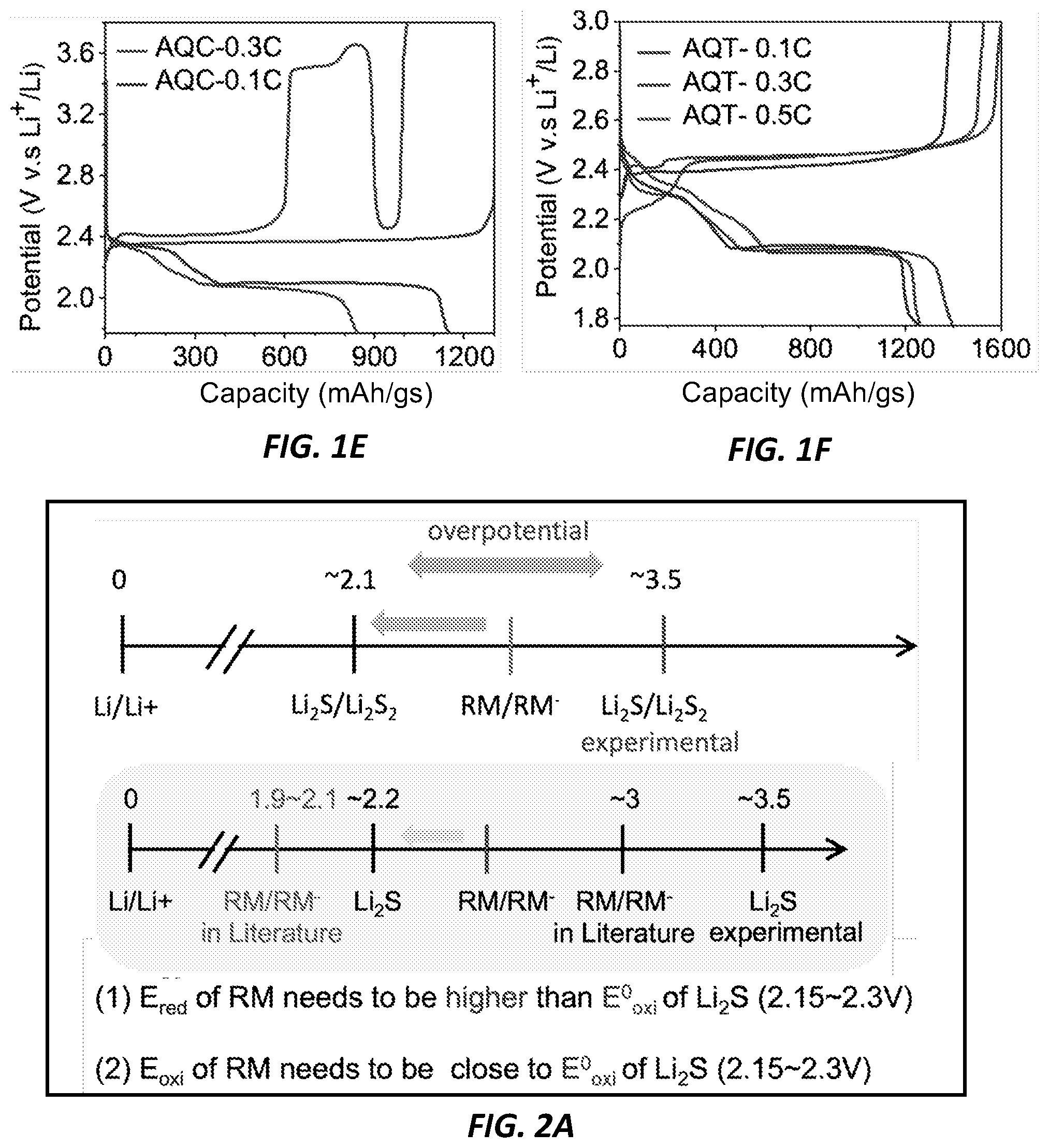
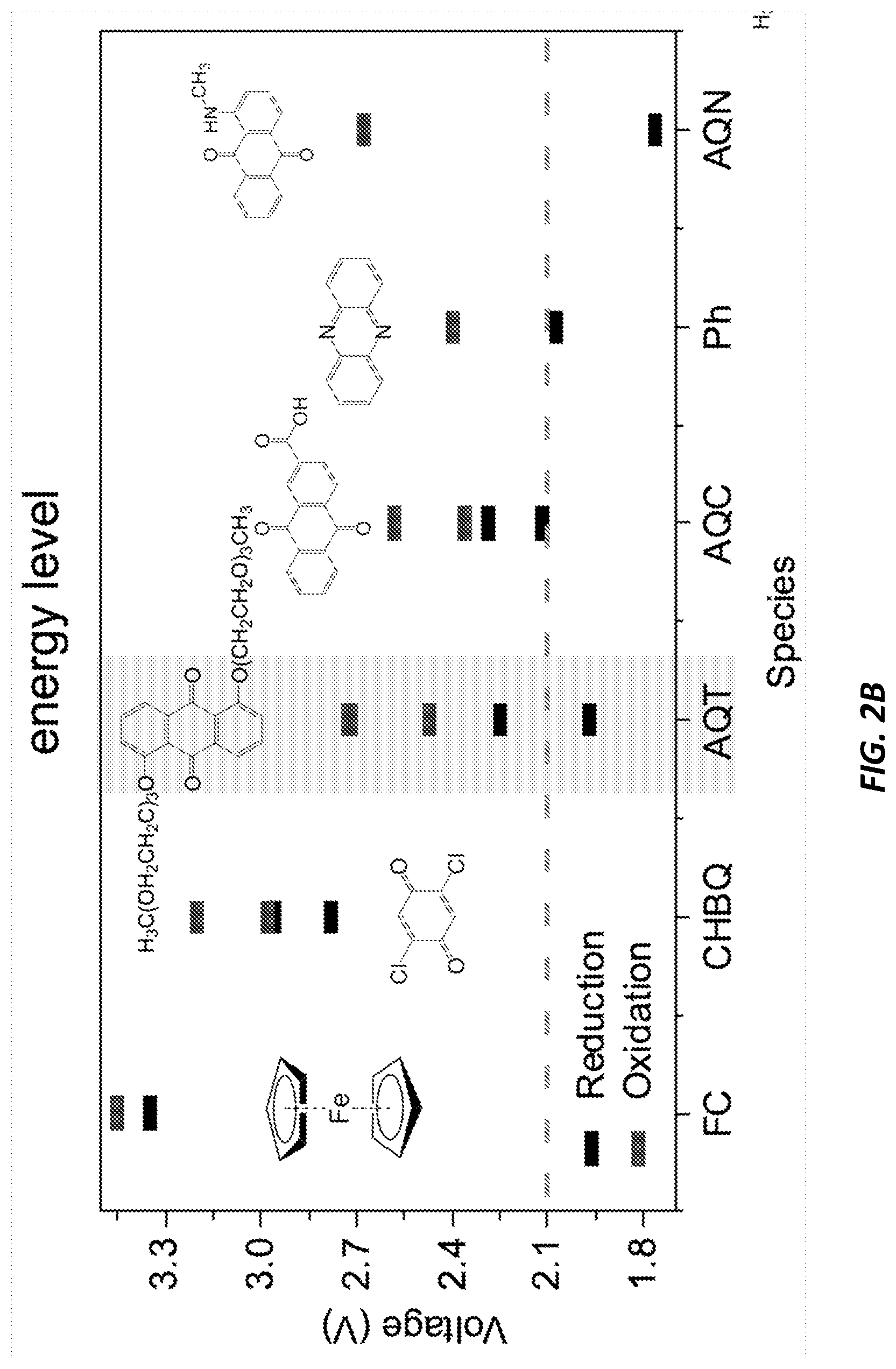

United States Patent
Application |
20210050624 |
Kind Code |
A1 |
Bao; Zhenan ; et
al. |
February 18, 2021 |
Rational design of redox mediator for fast and energy-efficient
charging of sulfur cathodes
Abstract
A battery electrolyte solution is that includes an organic
electrolyte solution, a lithium-sulfur (Li.sub.2S) compound,
soluble lithium polysulfide (Li.sub.2S.sub.x) microparticles, and a
redox mediator comprising a soluble quinone derivative.
Inventors: |
Bao; Zhenan; (Stanford,
CA) ; Cui; Yi; (Stanford, CA) ; Tsao;
Yuchi; (Palo Alto, CA) ; Lee; Min Ah; (Seoul,
KR) |
|
Applicant: |
Name |
City |
State |
Country |
Type |
The Board of Trustees of the Leland Stanford Junior
University |
Stanford |
CA |
US |
|
|
Family ID: |
1000005208460 |
Appl. No.: |
16/967851 |
Filed: |
February 6, 2019 |
PCT Filed: |
February 6, 2019 |
PCT NO: |
PCT/US19/16899 |
371 Date: |
August 6, 2020 |
Related U.S. Patent Documents
|
|
|
|
|
|
Application
Number |
Filing Date |
Patent Number |
|
|
62626936 |
Feb 6, 2018 |
|
|
|
62627458 |
Feb 7, 2018 |
|
|
|
Current U.S.
Class: |
1/1 |
Current CPC
Class: |
H01M 2300/0025 20130101;
H01M 10/0568 20130101; H01M 10/0567 20130101; H01M 2004/028
20130101; H01M 4/5815 20130101; H01M 10/052 20130101 |
International
Class: |
H01M 10/0567 20060101
H01M010/0567; H01M 10/0568 20060101 H01M010/0568; H01M 10/052
20060101 H01M010/052; H01M 4/58 20060101 H01M004/58 |
Claims
1) A battery electrolyte solution comprising: a) an organic
electrolyte solution; b) a lithium-sulfur (Li.sub.2S) compound; c)
soluble lithium polysulfide (Li.sub.2S.sub.x) microparticles; and
d) a redox mediator comprising a soluble quinone derivative.
2) The battery electrolyte solution of claim 1, wherein said
quinone derivative comprises
1,5-bis(2-(2-(2-methoxyethoxy)ethoxy)ethoxy)anthra-9,10-quinon
(AQT).
3) The battery electrolyte solution of claim 1, wherein said
soluble polysulfide Li.sub.2S.sub.x comprises a compound selected
from the group is consisting of Li.sub.2S.sub.2, Li.sub.2S.sub.4,
Li.sub.2S.sub.6, Li.sub.2S.sub.8, and Li.sub.2S.sub.x--S, wherein
x={2, 4, 6, 8}.
4) The battery electrolyte solution of claim 1, wherein a reduction
energy level of said redox mediator is higher than an oxidation
energy level of said battery electrode active material.
5) The battery electrolyte solution of claim 1, wherein a reduction
potential of said redox mediator is higher than an oxidation
potential of said battery electrode active material.
6) The battery electrolyte solution of claim 1, wherein a reduction
energy level of said redox mediator is higher than an oxidation
energy level of said redox mediator.
7) The battery electrolyte solution of claim 6, wherein a reduction
energy level of said redox mediator is higher than an oxidation
energy level of said Li.sub.2S compound.
8) The battery electrolyte solution of claim 6, wherein said is
reduction energy level is in a range of 2.15 V to 2.3 V.
9) The battery electrolyte solution of claim 6, wherein an
oxidation energy level of said redox mediator is higher than and
proximal to an oxidation energy level of said Li.sub.2S
compound.
10) The battery electrolyte solution of claim 9, wherein said
reduction energy level is in a range of 2.15 V to 2.3 V.
11) The battery electrolyte solution of claim 1, wherein a redox
potential of said quinone derivative ranges from 1.7 V to 3.2
V.
12) A Li--S battery comprising: a) an anode; b) a cathode; c) an
organic electrolyte solution; d) a current collector; e) a
lithium-sulfur (Li.sub.2S) compound; f) soluble lithium polysulfide
(Li.sub.2S.sub.x) microparticles; and g) a redox mediator
comprising a soluble quinone derivative.
13) The Li--S battery of claim 13, wherein said quinone derivative
comprises
1,5-bis(2-(2-(2-methoxyethoxy)ethoxy)ethoxy)anthra-9,10-quinon
(AQT).
14) The Li--S battery of claim 13, wherein said soluble polysulfide
Li.sub.2S.sub.x comprises a compound selected from the group
consisting of Li.sub.2S.sub.2, Li.sub.2S.sub.4, Li.sub.2S.sub.6,
Li.sub.2S.sub.8, and Li.sub.2S.sub.x--S, wherein x={2, 4, 6,
8}.
15) The Li--S battery of claim 13, wherein a reduction energy level
of said redox mediator is higher than an oxidation energy level of
said redox mediator.
16) The Li--S battery of claim 13, wherein a reduction energy level
of said redox mediator is higher than an oxidation energy level of
said Li.sub.2S compound.
17) The Li--S battery of claim 16, wherein said reduction energy
level is in a range of 2.15 V to 2.3 V.
18) The Li--S battery of claim 16, wherein an oxidation energy
level of said redox mediator is higher than and proximal to an
oxidation energy level of said Li.sub.2S compound.
19) The Li--S battery of claim 18, wherein said reduction energy
level is in a range of 2.15 V to 2.3 V.
20) The Li--S battery of claim 13, wherein a redox potential of
said quinone derivative ranges from 1.7 V to 3.2 V.
Description
FIELD OF THE INVENTION
[0001] The present invention relates generally to current
collectors. More particularly, the invention relates to activating
a sulfur cathode to near its equilibrium potential by adding a
small aromatic molecule in the electrolyte as a redox mediator
(RM).
BACKGROUND OF THE INVENTION
[0002] Lithium-sulfur (Li--S) batteries are a sustainable and
cost-effective solution for next generation energy storage to
support renewable energy integration due to the natural abundance
of sulfur. Li--S batteries can theoretically store an energy
density of 2500 Wh kg.sup.-1, a five-fold increase compared to
traditional lithium ion batteries (LIBs). Such exceptionally high
energy density is enabled by the reversible conversion reaction
between sulfur and lithium sulfide (Li.sub.2S) via a series of
lithium polysulfides intermediates (LiPSs, Li.sub.2S.sub.n,
2.ltoreq.n.ltoreq.8). However, significant challenges remain in
order to build practical Li--S batteries, which are mainly
attributed to the solubility of LiPSs in the electrolytes and the
insulating nature of both sulfur and Li.sub.2S. For examples, when
charging a Li.sub.2S electrode, a significant portion of each
particle is electrically isolated and can be oxidized at the
localized interface between the electrode/electrolyte with
sufficient charge transfer; therefore, the Li.sub.2S exhibits a
large overpotential and a limited reversible capacity that is
substantially lower than the theoretical value. Furthermore, the
dissolution-precipitation process yields insulating deposits of S
and Li.sub.2S, which passivate the active interface for the redox
reaction. Such evolution of the electrode architecture recurring
over cycling is thus considered one of the primary causes of
capacity fading in Li--S batteries together with internal
polysulfides shuttling. Consequently, Li--S cells have been shown
to have insufficient practical energy density, poor rate
capability, and limited cycle life, collectively restricting
commercial success of this system.
[0003] To address the aforementioned issues, considerable efforts
have focused on incorporating nanostructured conductors, such as
transition metals (Fe, Cu, Co), metal oxides, metal sulfides, metal
nitrides, metal carbides, carbon nanomaterials, and conductive
polymers. The electrochemically active area in the electrode
significantly improved through these approaches, and LiPSs
diffusion was further suppressed when appropriate physical
confinement was attained. However, this improvement was often
accomplished at the expense of the tap density with low active
material loadings and would require costly production processes.
Also, this approach does not necessarily prevent detachment of
active particles from a conductive support and passivation of redox
active sites upon long-term cycling during the solid-liquid-solid
transition. Employing chemical interactions between LiPSs and hosts
can better trap the sulfur species, but this effect is specific to
the host surfaces, raising concerns about practical viability of
requiring an ultrahigh-surface-area matrix. Therefore,
breakthroughs are still needed to develop a low cost, scalable and
reliable method to improve the practical performance of Li--S
batteries.
[0004] Introducing redox mediators (RMs) to the electrolyte
solution represents another effective strategy to address the
limited performance of Li--S batteries. This approach relies on
electrochemical oxidation of RMs in solution which can in turn
chemically oxidize the active material on the entire surface of the
particle. This additional charge transfer route beyond the
localized interface enables homogeneous and complete oxidation of
the electrode with a reduced overpotential. This approach has been
primarily explored in lithium-oxygen (Li--O.sub.2) batteries, which
suffer from the poor electronic conductivity of Li.sub.2O.sub.2.
Properly designed RMs have been shown to reduce the charge
overpotential of Li.sub.2O.sub.2 by less than 0.1 V and protect
both the electrolyte and carbon electrodes from degradation,
significantly improving the energy efficiency and cycling stability
of Li--O.sub.2 cells. In contrast, the research in utilizing RMs in
Li--S batteries is still in its infancy. Soluble LiPSs are known to
serve as an internal RM if available, but their transient nature
renders LiPSs unreliable in the cell at fully discharged or charged
states. So far, only metallocene has been confirmed to shuttle
electrons during Li.sub.2S oxidation, which can reduce the initial
charge potential of Li.sub.2S electrodes to 2.9 V. Lithium iodide
(LiI) was also reported to reduce the polarization, but later it
was found that it actually modified Li.sub.2S surfaces; regardless,
the charging occurs at 2.8 V, which still deviates from the
Li.sub.2S equilibrium potential. Hence, the lack of proper redox
chemistry has hindered the potential of employing RMs in Li--S
batteries when compared to Li--O.sub.2 batteries.
[0005] What is needed is a quinone redox chemistry to enable a new
RM for fast and stable cycling of Li--S batteries.
SUMMARY OF THE INVENTION
[0006] To address the needs in the art, a battery electrolyte
solution that includes an organic electrolyte solution, a
lithium-sulfur (Li.sub.2S) compound, soluble lithium polysulfide
(Li.sub.2S.sub.x) microparticles, and a redox mediator comprising a
soluble quinone derivative.
[0007] In one aspect of the invention, the quinone derivative
includes
1,5-bis(2-(2-(2-methoxyethoxy)ethoxy)ethoxy)anthra-9,10-quinon
(AQT).
[0008] In another aspect of the invention, the soluble polysulfide
Li.sub.2S.sub.x is a compound that includes Li.sub.2S.sub.2,
Li.sub.2S.sub.4, Li.sub.2S.sub.6, Li.sub.2S.sub.8, and
Li.sub.2S.sub.x--S, where x={2, 4, 6, 8}.
[0009] According to a further aspect of the invention, a reduction
energy level of the redox mediator is higher than an oxidation
energy level of the battery electrode active material.
[0010] In yet another aspect of the invention, a reduction
potential of the redox mediator is higher than an oxidation
potential of the battery electrode active material.
[0011] According to one aspect of the invention, a reduction energy
level of the redox mediator is higher than an oxidation energy
level of the redox mediator. In one aspect, a reduction energy
level of the redox mediator is higher than an oxidation energy
level of the Li.sub.2S compound. In another aspect, the reduction
energy level is in a range of 2.15 V to 2.3 V. In a further aspect,
an oxidation energy level of the redox mediator is higher than and
proximal to an oxidation energy level of the Li.sub.2S
compound.
[0012] According to one aspect, the reduction energy level is in a
range of 2.15 V to 2.3 V.
[0013] In one aspect of the invention, a redox potential of the
quinone derivative ranges from 1.7 V to 3.2 V.
[0014] According to one embodiment the invention includes a Li--S
battery having an anode, a cathode, an organic electrolyte
solution, a current collector, a lithium-sulfur (Li.sub.2S)
compound a soluble lithium polysulfide (Li.sub.2S.sub.x)
microparticles, and a redox mediator comprising a soluble quinone
derivative.
[0015] In one aspect of the current invention, the quinone
derivative includes
1,5-bis(2-(2-(2-methoxyethoxy)ethoxy)ethoxy)anthra-9,10-quinon
(AQT).
[0016] In another aspect of the invention, the soluble polysulfide
Li.sub.2S.sub.x includes a compound selected from the group
consisting of Li.sub.2S.sub.2, Li.sub.2S.sub.4, Li.sub.2S.sub.6,
Li.sub.2S.sub.8, and Li.sub.2S.sub.x--S, where x={2, 4, 6, 8}.
[0017] According to a further aspect of the invention, a reduction
energy level of the redox mediator is higher than an oxidation
energy level of the redox mediator.
[0018] In yet another aspect of the invention, a reduction energy
level of the redox mediator is higher than an oxidation energy
level of the Li.sub.2S compound. In one aspect, the reduction
energy level is in a range of 2.15 V to 2.3 V. In a further aspect,
an oxidation energy level of the redox mediator is higher than and
proximal to an oxidation energy level of the Li.sub.2S compound.
Here, the reduction energy level is in a range of 2.15 V to 2.3
V.
[0019] According to one aspect of the invention, a redox potential
of the quinone derivative ranges from 1.7 V to 3.2 V.
BRIEF DESCRIPTION OF THE DRAWINGS
[0020] FIG. 1A shows the typical voltage profile of a Li.sub.2S
electrode in the first cycle at 0.3C using monodispersed
microparticles (.mu.-Li.sub.2S, 1.26 .mu.m average diameter).
[0021] FIG. 1B shows the effective RMs solubilized in the
electrolyte shuttle electrons between current collectors and the
surfaces of isolated Li.sub.2S particles, which would have
otherwise remained inactive, according to the current
invention.
[0022] FIG. 1C presents cyclic voltammograms (CVs) of the RMs
versus lithium metal in the DOL/DME electrolyte. When compared with
FC, quinone derivatives showed more favorable redox potentials with
are batter matched to that of Li.sub.2S oxidation, according to the
current invention.
[0023] FIG. 1D shows the first cycle voltage profiles of Li.sub.2S
electrodes with different RMs at 0.3C, according to the current
invention.
[0024] FIGS. 1E-1F show a comparison of discharge rates for AQC vs.
AQT, according to the current invention.
[0025] FIGS. 2A-2B show diagrams of the overpotential and energy
levels relating to the RM's of the current invention.
[0026] FIGS. 3A-3C show, in the presence of AQT, the original
morphology of Li.sub.2S particles was virtually unchanged over 250
cycles, according to the current invention.
DETAILED DESCRIPTION
[0027] The development of Li.sub.2S electrodes is a crucial step
toward the industrial manufacturing of Li--S batteries, a promising
alternative to Li-ion batteries due to their projected two times
higher specific capacity. However, the high voltages needed to
activate Li.sub.2S electrodes, and the consequent electrolyte
solution degradation, represent the main challenge. Hence, the
discovery of effective catalyst is an important step to solving
this problem. According to the current invention, a series of
organic RM candidates are selected as a model system to identify
the key factor in determining the catalytic activities and in Li--S
cells. It is revealed that the level of oxidation potential,
ability to oxidize Li.sub.2S and solubility play an important role
for activating Li.sub.2S electrode. It is demonstrated that they
are critical in reducing the overpotential of the Li.sub.2S
electrode. Accordingly, the current invention provides a general
principle for designing feasible catalyst and report a RM, AQT,
with a remarkably low overpotential. The discovery leads to high
rate cycle stability (1C) can be achieved in 700 cycles with an
only decay rate of 0.034% per cycle.
[0028] The current invention provides an effective strategy to
activate a sulfur cathode near its equilibrium potential by adding
a small aromatic molecule in the electrolyte as a redox mediator
(RM).
[0029] The insulating nature of Li.sub.2S particles hinder the
charge transfer process and require intimate contact with current
collectors to become activated. This stringent limitation leads to
large first charge cycle polarization and decay of charge capacity.
To address these issues, a redox mediator (RM) can be used to
completely re-route charge transfer process between Li.sub.2S
particles and current collectors. RM can chemically oxidize
Li.sub.2S particles (Step 1 chemical reactions: RM become RM2-),
diffuse to current collectors and unload the electrons (Step 2
electrochemical reactions: RM2- become RM). Since RM can be
continuously regenerated, small quantities of RM can catalytically
shuttle electrons for entire cathodes efficiently. Notably, RM can
patrol the cathode compartment by free diffusion to identify and
react with Li.sub.2S particles, not in proper contact with current
collectors. Hence, RM fundamentally resolves issues imposed by
insulating Li.sub.2S particles. Spontaneous chemical oxidation of
Li.sub.2S particles by RM necessitates the reduction potential of
RM higher than Li.sub.2S oxidation potential (2.15V). Further,
since RM is responsible for the release of electrons to current
collectors, the cathode oxidation potential is now determined by
oxidation potential of RM (the potential from RM2- to RM).
Therefore, selecting RM with oxidation potential close to 2.15 V
can substantially reduce the first charge overpotential
(.about.3.5V). Moreover, by introducing two triethylene glycol
monomethyl ether groups on the RM core, the resulting molecule
(denoted as AQT) shows substantial improvement in the solubility,
resulting in outstanding charge potential improvement and cycle
stability.
Applications:
[0030] 1. Traditional approaches mostly require fabrication of
complicated electrodes structures, which could adversely increase
battery production costs. The redox mediator (RM) of the current
invention could be easily blended into the electrolyte and
dropcasted on the electrodes. Thereby, it is foreseen that the RM
is a promising material that permits scalable production of high
performing Li2S cathodes. [0031] 2. The energy design principle is
universal, which can be applicated in all other unconducive battery
materials.
[0032] Because most research is focused on producing the
nanoparticle Li.sub.2S or creating an encapsulating structure with
conductive materials to solve the insulating nature of sulfur
materials, the synthesis is too complicated. In one aspect of the
invention, the mediator approach is less complex--just dissolve the
RM in the electrolyte without any synthetic procedure of electrode
materials.
[0033] According to the current invention, the approach is
universal for Li--S batteries that does not change either the
electrolytes or the electrode of the battery system. In essence,
the current invention provides an effective, simple and universal
approach that can easily implant any method that has been provided
in the Li--S battery field.
[0034] Many strategies have been proposed to improve first charge
cycling performance. First, many efforts have been dedicated to
improving contact of Li.sub.2S particles with conducting materials
by blending or encapsulating Li.sub.2S with high-surface-area
conductive material. Common conductive materials include transition
metal (Fe, Cu, Co), transition metal oxide or carbide, carbon
materials (e.g., graphene and carbon nanofibers) and conductive
polymers. However, the first charge overpotential is still limited
to above 2.8 V.
[0035] Second, incorporation of RM is another promising approach.
However, all literature reported RMLi.sub.2S cathodes still show
first charge overpotential above 3V. According to the current
invention, AQT disclosed herein is the only RM that can
significantly reduce overpotential down to 2.4V up to 0.5 C charge
rate, and outperforms current literature reported counterparts. An
enhanced cycle stability is also demonstrated by AQTLi.sub.2S
electrode. The cycle retention has improved from 60% to 81% in 350
cycles compared to the bare Li.sub.2S electrode. Moreover, only a
few studies successfully display high rate 1C cycle stability over
1000 cycles, and electrode encapsulation is needed. Nevertheless,
provided herein the cycle retention of 76% over 700 cycles can be
successfully maintained. Furthermore, AQT could successfully
resolve first charge overpotential in high mass loading Li.sub.2S
cathodes (3-6 mg/cm.sup.2) and maintain high cycling stability up
to 100 cycles (900 mAh/gs).
[0036] According to the current invention, a rational design
principle has been created for the sulfur cathode, including
potential selection rule, solubility effect for different
functional group tuning. This leads to finding a chemical structure
that is the most suitable RM for sulfur battery cathode.
[0037] Due to the insulating nature of Li.sub.2S, hinders the
charge transfer process between Li.sub.2S and current collector,
causing large polarization in the first cycle, accumulation of
unreacted Li.sub.2S during cycles. The thick and inhomogeneous
Li.sub.2S would eventually block the cathode and cause a death
cell. Besides, because of repeated dissolution-deposition process
of polysulfide, Li.sub.2S loses intimate contact with current
collector and therefore becomes inactive (isolated Li.sub.2S) in
the subsequent cycling. But with adding small molecule (RM) to the
electrolyte that reversibly accept and donate electrons at known
potential, it can completely re-route the electron transfer process
between Li.sub.2S and current collector by shuttling electrons
between Li.sub.2S and current collector continuously, facilitating
charge transfer kinetics throughout the electrode and making the
isolated Li.sub.2S active through cycling.
[0038] Turning now to a more specific discussion, lithium-sulfur
(Li--S) batteries charge by oxidizing solid lithium sulfide
(Li.sub.2S) into sulfur (S.sub.8) through soluble lithium
polysulfide intermediates (Li.sub.2S.sub.x), enabling a high
theoretical specific capacity of 1675 mAh g.sub.s.sup.-1. However,
the insulating nature of sulfur and Li.sub.2S results in large
polarization and low sulfur utilization while the soluble
polysulfides lead to internal shuttle upon charge and discharge.
Furthermore, the redox reaction via the dissolution-precipitation
route destroys the electrode architecture by passivating the active
interface responsible for the redox reaction, and thus the
performance of Li--S batteries deteriorates with cycling. Disclosed
herein is the redox chemistry of a quinone derivative to realize
efficient, fast, and stable operation of Li--S batteries using
Li.sub.2S microparticles. By adding a quinone derivative with
tailored properties (e.g. oxidation potential, solubility, and
electrochemical stability in the electrolyte) to an electrolyte as
a redox mediator, initial charging of Li.sub.2S electrodes occurs
below 2.5 V at a 0.5C rate, and the subsequent discharge capacity
is as high as 1300 mAh g.sub.s.sup.-1. Moreover, deposition of dead
Li.sub.2S, which was the primary cause of increasing polarization
and decreasing reversible capacity of Li--S batteries upon cycling,
is effectively prevented with the addition of the redox
mediator.
[0039] Here, quinone redox chemistry is used to design a new RM for
fast and stable cycling of Li--S batteries. Through rational tuning
of the redox potential, stability, and solubility of quinones by
molecular engineering, it is successfully demonstrated that the
quinone redox can facilitate the initial oxidation of Li.sub.2S
below 2.5 V at a fast rate of 0.5C. The continuing effectiveness of
the quinone-based RM beyond the first cycle was confirmed with
observation of the minimal polarization and improved capacity
retention of Li--S cells over prolonged cycles. Importantly, when
cycled with the RM, the Li.sub.2S electrode maintained its original
morphology over 250 cycles, suggesting a unique mechanism that
enables controlled deposition of Li.sub.2S and sulfur instead of
random deposition. Thus, minimal overpotential and high capacity
continued over prolonged cycling. Because of these collective
attributes, a high mass loading electrode of 6 mg cm.sup.-2
Li.sub.2S was used to successfully demonstrate charging below 2.5 V
with a reversible capacity of 952 mAh g.sub.s.sup.-1. This
effective strategy of using tailored RMs in sulfur redox chemistry
to improve both reaction kinetics and stability is the key to
obtaining high practical power and energy density over prolonged
cycling for the practical implementation of Li--S batteries.
[0040] Turning now to a discussion of large overpotentials for
Li.sub.2S cathodes, due to poor electronic conductivity, Li.sub.2S
electrodes are difficult to activate. The oxidation reaction only
occurs at the localized regions of the active particles directly
interfacing electron and ion transfer channels. This mechanism thus
requires a large overpotential, and the cell exhibits a specific
capacity much lower than the theoretical value. FIG. 1A shows the
typical voltage profile of a Li.sub.2S electrode in the first cycle
at 0.3C using monodispersed microparticles (.mu.-Li.sub.2S, 1.26
.mu.m average diameter), and the conventional electrolyte for Li--S
batteries [i.e., 1 M lithium bis(trifluoromethanesulfonyl) imide in
dioxolane/dimethoxyethane (DOL/DME) with 2 wt % LiNO.sub.3]. The
electrolyte to Li.sub.2S ratio was 28 .mu.l mg.sup.-1 which should
be considered a flooded electrolyte condition. Upon charging, an
overshoot as high as 3.6 V followed by a quick voltage drop to 2.6
V, then a high charge potential over 3.6 V was observed. A high
charge cut-off voltage of 3.8 V, which exceeds the stability limit
of ethereal electrolytes, was applied in an attempt to further
activate Li.sub.2S; however, the discharge capacity was still less
than 800 mAh g.sub.s.sup.-1, indicating less than 50% sulfur
utilization. At a lower rate of 0.1C, the overpotential was
reduced, permitting a lower charge cut-off voltage of 3.0 V;
however, the discharge capacity remained insufficiently low,
indicating a substantial amount of inactive Li.sub.2S (52% sulfur
utilization).
[0041] Effective RMs solubilized in the electrolyte shuttle
electrons between current collectors and the surfaces of isolated
Li.sub.2S particles, which would have otherwise remained inactive
(see FIG. 1B). During charging, the oxidized RMs with a redox
potential higher than that of Li.sub.2S can chemically oxidize
Li.sub.2S over the entire surface interfacing with the electrolyte
and diffuse to current collectors where they are then
electrochemically re-oxidized. Consequently, the charge voltage
reflects the redox potential of the RM. Thus, the ideal redox
potential of RMs would be slightly higher than the equilibrium
potential of Li.sub.2S (.about.2.15 V vs Li.sup.+/Li) to minimize
the hysteresis between charging and discharging in order to
maximize energy efficiency. However, RMs in literature including
ferrocene (FC) and decamethylferrocene exhibit significantly higher
redox potentials at 3.4 V and 2.9 V vs Li.sup.+/Li, respectively.
To design RMs with better matched redox potentials, the redox
activity of quinones are disclosed herein. The redox potentials of
quinone molecules range from 1.7 V to 3.2 V vs. Li.sup.+/Li
depending on the molecular structure. It is rationalized here that
anthraquinone (AQ) derivatives possess lower redox potential than
benzoquinone (BQ) derivatives due to the electron-rich benzene
rings. Further, the molecular structure of AQ is tailored to
control the solubility in DOL/DME electrolyte and stability to
maximize RM efficiency.
[0042] Anthraquinone-2-carboxylic acid (AQC) and
2,5-dichloro-1,4-benzoquinone (DCBQ) are selected as RMs to examine
electrochemical properties. FIG. 1C presents cyclic voltammograms
(CVs) of the RMs versus lithium metal in the DOL/DME electrolyte.
When compared with FC, quinone derivatives showed more favorable
redox potentials with are batter matched to that of Li.sub.2S
oxidation. In particular, AQC is preferred to facilitate Li.sub.2S
oxidation with minimal polarization. However, with continued
cycling, the AQC had limited electrochemical stability in the
operating condition of Li--S cells. Moreover, AQC had limited
solubility (<20 mM) in the DOL/DME electrolyte, further limiting
the usable RM quantity in the cell. To achieve a better RM polar
substituents (triethylene glycol monomethyl ether) were introduced
to the AQ center to yield
1,5-bis(2-(2-(2-methoxyethoxy)ethoxy)ethoxy) anthra-9,10-quinon
(AQT), which exhibits similar redox potentials to AQC (FIG. 1C).
AQT showed improved solubility (>500 mM) and markedly improved
cycling stability compared to AQC, rendering the molecule more
reliable as a RM.
[0043] The first cycle voltage profiles of Li.sub.2S electrodes
with different RMs at 0.3C are shown in FIG. 1D. The same electrode
and electrolyte condition used in FIG. 1A was employed for a
comparative study except that 80 mM of RM was added to the
electrolyte. The molar ratio of Li.sub.2S to RM was fixed to 10:1.
In accordance with current invention rationales, AQT demonstrated
exceptional performances when compared to other RMs. While the
addition of both AQC and AQT prevents the initial overshoot upon
charging, only AQT can oxidize Li.sub.2S at a constant potential
below 2.5 V throughout the charging process. Remarkably, the cell
with AQT exhibited much higher discharge capacity of 1402 mAh
g.sub.s.sup.-1, corresponding to 85% sulfur utilization, than the
cell with AQC. Even under the condition where AQC and AQT were both
fully soluble (20 mM) (see FIGS. 1E-1F for a comparison of
discharge rates), the cell with AQT showed a higher discharge
capacity (810 mAh g.sub.s.sup.-1) than that with AQC (482 mAh
g.sub.s.sup.-1), which may be due to the deteriorating stability of
AQC during regeneration. On the other hand, DCBQ and FC showed
limited improvement in promoting Li.sub.2S oxidation as expected
since their redox potentials are significantly higher than 2.1 V.
The initial voltage overshoot decreased but remained above 3.0 V
and 3.4 V in for DCBQ and FC, respectively, which is consistent
with their redox potentials. Once LiPSs were formed and available
as the additional RM in the electrolyte, the voltage dropped to 2.6
V. As LiPSs were exhausted, the voltage rose back to the redox
potential of DCBQ and FC, respectively, which was then followed by
another dip in the profile by forming additional LiPSs. Given this
insufficient activation of the Li.sub.2S electrode, the resulting
discharge capacity did not improve considerably in either system
when compared to the cell without RM.
[0044] From the energy efficiency and discharge capacity of the
various RMs, it is determined that low polarization and high
Coulombic efficiency lead to high energy efficiency. According to
the current invention, the Li.sub.2S cell with AQT showed the
highest discharge capacity (1402 mAh g.sub.s.sup.-1), lowest
average charge potential (<2.5 V) and highest Coulombic
efficiency (87%), thus exhibiting the highest energy efficiency
among all tested RMs. Taken together, AQT with the desired redox
potential, high solubility in the electrolyte and superior cycling
stability is the most effective in promoting charge transport of
Li.sub.2S cathodes among all the tested systems.
[0045] In one example, with 10 mM of AQT in the electrolyte, where
the molar ratio between Li.sub.2S and AQT is 80 to 1, the
overpotential during the first charging cycle can be effectively
reduced. Nevertheless, as the AQT concentration increases, the
reversible capacity and Coulombic efficiency increase. Thus, either
80 mM or 160 mM of AQT depending on the electrode mass loading in
the following study to assure improved performance. When the
electrolyte amount was also decreased from 20 .mu.l to 10 .mu.l,
which corresponds to the electrolyte/Li.sub.2S of 14 .mu.l
mg.sup.-1, the Li.sub.2S electrode can be still charged at 2.5V.
Note that redox cycling of quinone is also effective in oxidizing
ball-milled commercial Li.sub.2S with a more heterogeneous size
distribution than the .mu.-Li.sub.2S.
[0046] Notably, AQT performs better than Li.sub.2S.sub.8, the
internal RM spontaneously formed during conventional sulfur redox
processes. When adding 80 mM Li.sub.2S.sub.8 as a RM to the DOL/DME
electrolyte, the charge capacity obtained below 2.5 V is less than
400 mAh g.sub.s.sup.-1 at 0.3C, which is three-fold less than AQT.
This result confirmed the need for a RM better than Li.sub.2S.sub.8
to minimize polarization during charging.
[0047] Next, the maximum C rate achieved was compared with various
reported strategies using Li.sub.2S electrodes and the
corresponding first average charging voltage. Methods such as
mixing Li.sub.2S with nanostructured conductors like carbon, metals
sulfides, and polymers still exhibited oxidation potentials of 2.8
V, 3.3 V, and 3.5 V, respectively, even at a slow rate (<0.1C),
simultaneously suffering from process complexity and lower active
material loadings. Likewise, the previous best performing additives
LiI and FC exhibited first charge potentials of 2.8 V at 0.05C and
2.9 V at 0.2C, respectively, which are far above the equilibrium
potential of Li.sub.2S. In comparison, AQT can facilitate Li.sub.2S
oxidation at voltages as low as 2.45 V even at a high current
density of 0.5C, which demonstrated that this system has
exceptionally fast charge transfer kinetics. The performance
comparison is more reasonable when the critical cell parameters
including areal mass loading, active content, and
electrolyte/active ratio are considered in parallel. Note that the
charge overpotential and the subsequent discharge capacity in this
example study is remarkable despite using large particles, a high
active content, and a comparable electrolyte to Li.sub.2S
ratio.
[0048] FIGS. 2A-2B show a diagrams of the overpotential and energy
levels relating to the RM's of the current invention.
[0049] Turning now to the chemical reaction between AQT and
Li.sub.2S, the voltage profiles of half cells using 80 mM AQT in
DOL/DME electrolyte (20 .mu.l) with and without a Li.sub.2S
electrode, exhibit capacities of 0.612 mAh and 0.059 mAh,
respectively. Given that the charge voltage of the Li.sub.2S
electrode overlaps with the voltage range of AQT at 2.4 V, the
charge process should involve the direct electrochemical oxidation
of AQT. In the galvanotactic discharge and charge of AQT (as a
catholyte), it is confirmed that there exists stable reduction and
oxidation of AQT with 80% capacity retention over 200 cycles and
negligible redox shuttling of AQT with Coulombic efficiency higher
than 98% throughout cycling. This result validates that AQT can
continuously be reduced and oxidized in the presence of a lithium
metal anode without significant degradation of the RM on the Li
metal surface.
[0050] The spontaneous chemical reaction of Li.sub.2S oxidation by
AQT was investigated by X-ray photoelectron spectroscopy (XPS). To
probe changes in the oxidation state of Li.sub.2S when mixed with
AQT in its oxidized state, the S2p binding energy of the Li.sub.2S
and AQT mixture (5:1 molar ratio) in the DOL/DME solvent were
measured. The result was compared to that of blank Li.sub.2S and
chemically synthesized Li.sub.2S.sub.4. The S2p spectrum of
Li.sub.2S.sub.4 shows two pairs of doublets at 161.20/162.4 eV and
162.8/163.9 eV, which corresponds to the terminal (S.sub.T.sup.-1)
and bridge (S.sub.B.sup.0) S atoms, respectively, in addition to
minor contributions from the unreacted Li.sub.2S at
159.67.20/160.85 eV. Similarly, the mixture of Li.sub.2S and AQT
exhibited significant contribution at higher S2p binding energies
than pristine Li.sub.2S. This arises from the oxidation of
Li.sub.2S by AQT, and thus proves the spontaneous charge transfer
between Li.sub.2S and AQT.
[0051] The spontaneous chemical reaction of Li.sub.2S oxidation by
AQT is better understood by analyzing the reaction product. Since
the reaction product simultaneously forms when we mix the Li.sub.2S
electrode and the AQT-containing electrolyte to fabricate the half
cell, the product is thus able to be electrochemically analyze by
directly discharging the half cell. The discharge profile of the
half cell with a mixture of Li.sub.2S and 160 mM AQT was compared
with the cell without Li.sub.2S and only having 160 mM AQT. Note
that no discharge capacity would be exhibited when directly
discharging pristine Li.sub.2S. From the mixture, on the other
hand, it is observed that a plateau exists at 2.25 V corresponding
to the electrochemical reduction of the AQT.sup.-, followed by a
plateau at 2.1 V corresponding to the electrochemical reduction of
Li.sub.2S.sub.x. Since the profile does not show the first
reduction plateau of AQT at 2.45 V, it is confirmed that all of the
AQT has been chemically converted into AQT.sup.- when mixed with
Li.sub.2S. Simultaneously, chemical oxidation of Li.sub.2S into
Li.sub.2S.sub.x should occur. The discharge capacity is identical
to that of pure AQT, indicating that the total amount of charge is
retained and no side reaction occurs other than this process.
[0052] Further evidence of the charge transfer process between AQT
and Li.sub.2S was obtained with cyclic voltammetry. A cathodic
sweep was conducted followed by an anodic sweep of AQT in DOL/DME
electrolyte while gradually adding Li.sub.2S.sub.4. The second
oxidation peak at 2.6 V (O2) showed significant current responses
upon addition of Li.sub.2S.sub.4, while the first oxidation peak
(O1) showed negligible changes. This result indicates that the
concentration of AQT.sup.- to be oxidized at 2.6 V increases as the
polysulfide concentration in the electrolyte increases due to the
chemical regeneration of AQT.sup.- from AQT. It is hypothesize that
the chemical regeneration of AQ.sup.- from AQ is promoted by
Li.sub.2S.sub.x (x<4), which can be formed during the previous
cathodic sweep by reducing Li.sub.2S.sub.4. (for details, see
Supplementary Note 1).
[0053] Turning now to the universal improvement in sulfur
electrochemistry by AQT, the effectiveness of AQT to improve the
Li--S battery operation beyond the first cycle was confirmed over
continued cycling. During 10 cycles at 0.1C, the blank Li.sub.2S
electrode showed repeated overshooting in the initial stage of
charging and the capacity continuously decreased to 400 mAh
g.sub.s.sup.-1. In contrast, with AQT, no voltage overshoot was
observed during charging, and 96% of the capacity at the second
cycle was retained in the 10th cycle. In the study, enlarged dQ/dV
curves displayed two consistent reduction peaks from AQT over 10
cycles which were not observed in the pristine Li.sub.2S cell,
showing continuous redox activity of AQT over prolonged
cycling.
[0054] To validate the effect of AQT to promote the sulfur
electrochemistry generally, cells using a Li.sub.2S.sub.8 catholyte
instead of a Li.sub.2S electrode were fabricated. The cells were
first discharged to reduce Li.sub.2S.sub.8 to Li.sub.2S and
comparable capacities were obtained regardless of the AQT presence
because pristine AQT does not participate in the reduction of
Li.sub.2S.sub.8. Then, the cell charging with AQT showed a slightly
lower overpotential when compared to the blank cell, and the
subsequent discharge capacity was much higher in the presence of
AQT. Note that the increased capacity (307 mAh g.sub.s.sup.-1) in
the second cycle cannot be fully accounted for the capacity
contribution from AQT indicating more Li.sub.2S can be activated
with AQT in the Li.sub.2S.sub.8 catholyte cells as well. Upon
prolonged cycling, the effect of AQT became prominent. Am average
charge and discharge voltage of the Li.sub.2S.sub.8 catholyte cells
with AQT at rates of 0.1C, 0.3C and 0.5C were compared to the blank
Li.sub.2S.sub.8 cell at 0.1C. The reduced polarization by AQT
remarkably persisted over 200 cycles, demonstrating consistent
charge transport kinetics over many cycles, outperforming the blank
cells.
[0055] For improving cycling stability of Li--S batters by AQT, it
is found that adding AQT significantly enhances the cycling
stability of Li--S batteries. At a current density of 1C, the
capacity of 850 mAh g.sub.s.sup.-1 was retained after 500 cycles in
the presence of AQT, whereas the capacity of the bare Li.sub.2S
cell decayed drastically to 225 mAh g.sub.s.sup.-1 within 10
cycles. At a lower rate of 0.5C, the capacity retention was still
considerably better with AQT. This cycling stability is exceptional
because it was achieved without specialized processing such as
confinement or encapsulation of the sulfur species. The energy
efficiency and Coulombic efficiency is also improved by adding AQT.
It is also important to note that AQT does not accelerate the
self-discharge discharge of Li--S batteries; but it prevents the
capacity decrease due to the sulfur loss in later cycles,
suggesting that shuttling of RM to the Li metal anode is
negligible.
[0056] The capacity decay of Li--S batteries is attributed to the
loss of active material due to the soluble intermediates and the
propagation of electrochemically inactive portion by pulverisation
and random deposition of insulating species during cycling.
Therefore, to understand the origin of the improved cycle life with
AQT, the effects of AQT on both polysulfide dissolution and
Li.sub.2S morphology evolution were explored.
[0057] Examined first was the solubility of representative LIPS
species (Li.sub.2S.sub.4 and Li.sub.2S.sub.8) in DOL/DME upon AQT
introduction. Polysulfide solutions (20 mM) were prepared as
references, and an equivalent molar amount of AQT was added to the
polysulfide solutions to prepare the mixtures. After filtering the
solution to remove any particles, inductively coupled plasma
optical emission spectrometry (ICP-OES) was used to quantify the
total concentration of sulfur and lithium in the liquid phase. The
Li.sub.2S.sub.4 and AQT mixture contained only 25% of the expected
sulfur content from the original pristine Li.sub.2S.sub.4 solution,
and the Li.sub.2S.sub.8 and AQT mixture also contained less than
55% of the sulfur content from the pristine Li.sub.2S.sub.8
solution. Therefore, introducing AQT decreased the amount of
soluble sulfur species in the electrolyte. The decreased LiPSs
solubility is attributed to the binding interaction between AQT and
LiPSs.
[0058] Next, the morphology evolution of Li.sub.2S electrodes over
many cycles was monitored. The Li.sub.2S electrodes tested with and
without AQT were retrieved after the first and 250th cycles,
respectively, and examined by scanning electron microscopy (SEM).
In accordance with the previous study under the conventional
condition suffering from the recurring solid-liquid-solid
transition, randomly deposited sulfur species that are different
from the initial morphology were observed in the blank Li.sub.2S
electrode regardless of the cycle number and state of the charge.
Such uneven accumulation of thick sulfur/Li.sub.2S layers observed
in SEM would block the charge transfer across the
electrode/electrolyte interfaces and thus cause poor reaction
kinetics and capacity decay. Nevertheless, in the presence of AQT,
the original morphology of Li.sub.2S particles was virtually
unchanged over 250 cycles (FIGS. 3A-3C). Such phenomenon
consistently was observed from multiple spots over the entire
electrode. This controlled deposition of the solid species was
identified as a critical feature to achieve long term cycling to
maintain the efficient charge transfer kinetics of the original
porous electrodes throughout the operation. The consistent
morphology over prolonged cycling that accompanies the repeated
solid-liquid-solid transition between Li.sub.2S and S cannot be
fully account for by the homogeneous oxidation over the Li.sub.2S
surface in the presence of AQT.
[0059] It is hypothesized that AQT can potentially alter
LiPS-solvent interactions and determine sulfur speciation, as
evidenced by the reduced solubility of LIPS with AQT, which in turn
induces preferred deposition of Li.sub.2S and S on the surfaces of
active particles.
[0060] Further conducted was ex situ X-ray spectromicroscopy with
the Li.sub.2S electrodes in the charged state that had been cycled
over 200 times. The maps were collected at multiple spots on each
sample (as indicated with area #1 and #2) at energies of 2470.8,
2472.6, 2473.6, and 2476.4 eV, in order to differentiate between
Li.sub.2S, polysulfide, and elemental sulfur species. After cycling
for 200 times in the presence of AQT, the fully charged electrode
showed a homogeneous sulfur distribution and a trace of Li.sub.2S
throughout the electrode. In sharp contrast, when cycled without
AQT, the Li.sub.2S electrode displayed a localized region of sulfur
with exceptionally high intensity (6-40 times higher than other
regions) in the elemental sulfur map while also showing significant
intensities from unreacted Li.sub.2S in the Li.sub.2S map. This is
consistent with our SEM result showing heterogeneous conversion of
sulfur during cycling. In sharp contrast, Thus, the results verify
homogenous and complete conversion of sulfur over the entire
electrode in the presence of AQT, which is highly desirable for
improved energy density and cycle life. Due to the complex nature
of sulfur speciation, further studies are needed to specify the
sulfur redox mechanism with AQT by real-time monitoring of chemical
and structural evolution of the electrode.
[0061] The above results suggest that AQT may effectively resolve
poor charge transfer and heterogeneity in high mass loading
electrodes. Thick Li.sub.2S electrodes were tested with areal
Li.sub.2S loading of 4 and 6 mg cm.sup.-2 in the presence of AQT.
The first charge plateau remained below 2.6 V at 0.05C throughout
the charging process, and more than 950 mAh g.sub.s.sup.-1 of
discharge capacity was obtained in both electrodes. This supports
the hypothesis that the redox mediating function of AQT effectively
facilitates Li.sub.2S oxidation even in thick electrodes with an
electrolyte to Li.sub.2S ratio of 12.5 .mu.l mg.sup.-1. When cycled
at 0.1C over 100 cycles, the 4 mg cm.sup.-2 electrode still
exhibited a discharge capacity of 863 mAh g.sub.s.sup.-1 with an
average Coulombic efficiency of 93.7% and the 6 mg cm.sup.-2
electrode exhibited 606 mAh g.sub.s.sup.-1 with an average
Coulombic efficiency of 92.8%. Notably, the above results were
obtained by using .mu.-Li.sub.2S (70 wt % of active content)
without adopting any nanostructuring or hosts for the first time.
This highlights the viability of using AQT as a practical strategy
to dramatically improve the performance of Li.sub.2S electrodes for
Li--S batteries. Nevertheless, when combined with optimized
electrode architectures, further improvements in the performance
and cell parameters (e.g. mass loading and electrolyte amount)
would be feasible and would truly translate our fundamental
discovery to real applications.
[0062] Also confirmed was the charge transfer kinetics in the
Li.sub.2S cathode cycled with AQT is superior to that of the
Li.sub.2S.sub.8 catholyte. A comparison of multiple charge profiles
were taken over prolonged cycling of the Li.sub.2S cathode with AQT
against Li.sub.2S.sub.8 catholyte having the equivalent sulfur
loading of 3 mg cm.sup.-2 and the active content of 70%.
Surprisingly, it was found that voltage profiles significantly
evolved over time in the catholyte system whereas the profiles of
the AQT-added Li.sub.2S cathode remained unchanged for more than
100 cycles. When comparing the average voltage of charge and
discharge curves over cycling for those two conditions, the results
were reliably consistent and lower polarization was observed in the
Li.sub.2S electrode with AQT, highlighting stable and efficient
sulfur redox cycling enabled by AQT.
[0063] The present invention has now been described in accordance
with several exemplary embodiments, which are intended to be
illustrative in all aspects, rather than restrictive. Thus, the
present invention is capable of many variations in detailed
implementation, which may be derived from the description contained
herein by a person of ordinary skill in the art. All such
variations are considered to be within the scope and spirit of the
present invention as defined by the following claims and their
legal equivalents.
* * * * *