U.S. patent application number 16/200988 was filed with the patent office on 2019-09-26 for alkylamine functionalized metal-organic frameworks for composite gas separations.
This patent application is currently assigned to THE REGENTS OF THE UNIVERSITY OF CALIFORNIA. The applicant listed for this patent is THE REGENTS OF THE UNIVERSITY OF CALIFORNIA. Invention is credited to Deanna M. D'Alessandro, Jeffrey R. Long, Thomas M. McDonald.
Application Number | 20190291074 16/200988 |
Document ID | / |
Family ID | 48141364 |
Filed Date | 2019-09-26 |


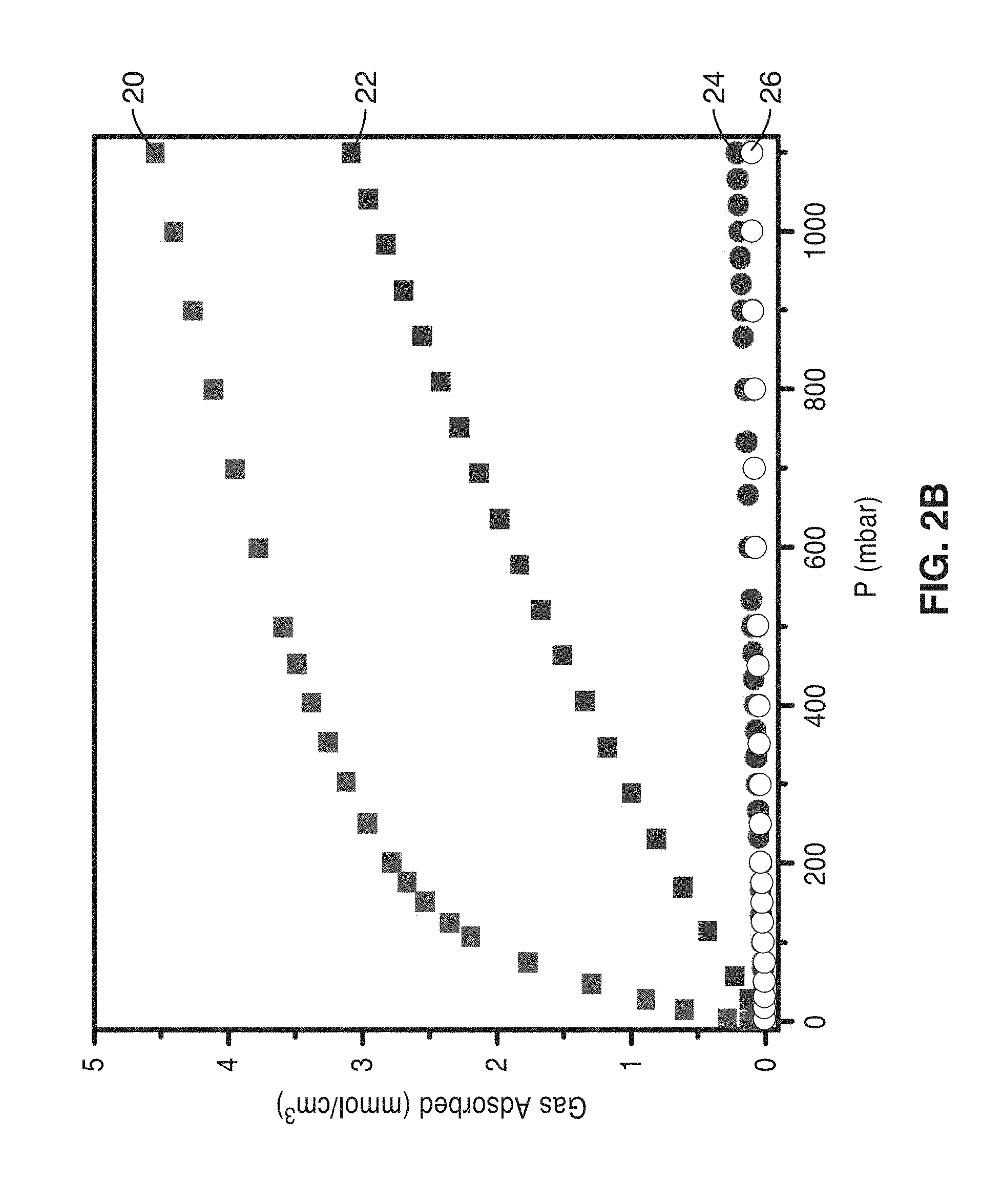



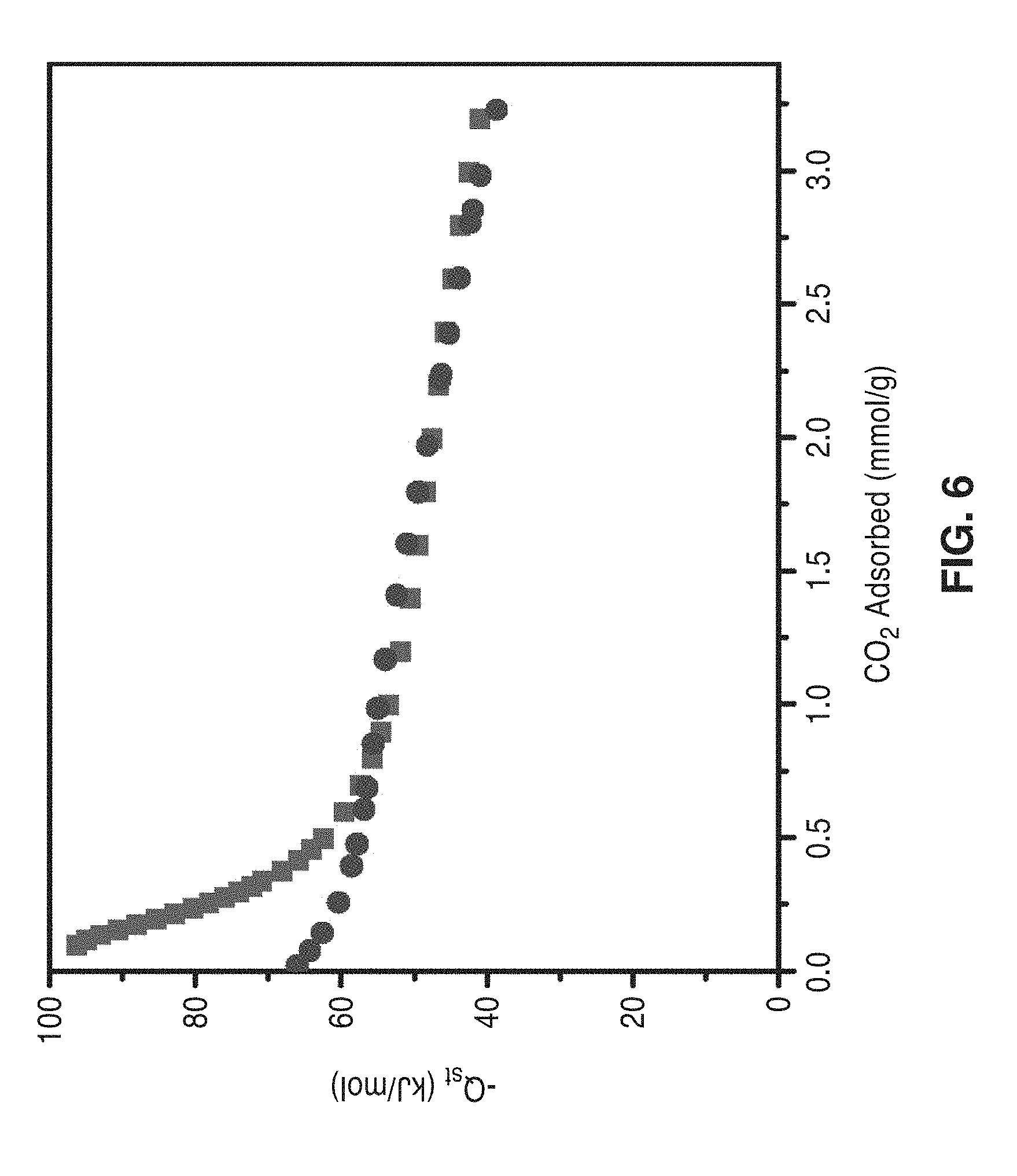
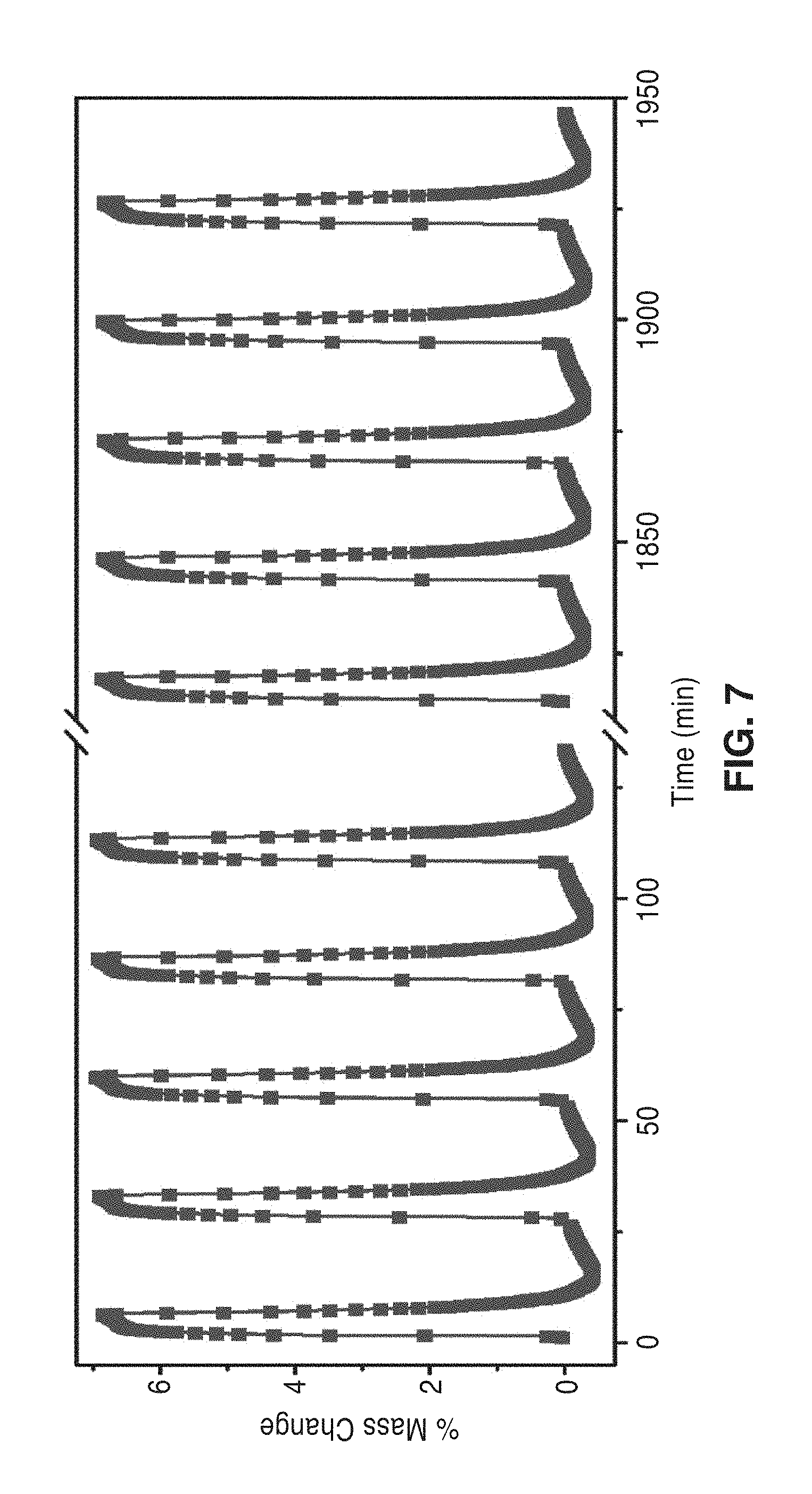

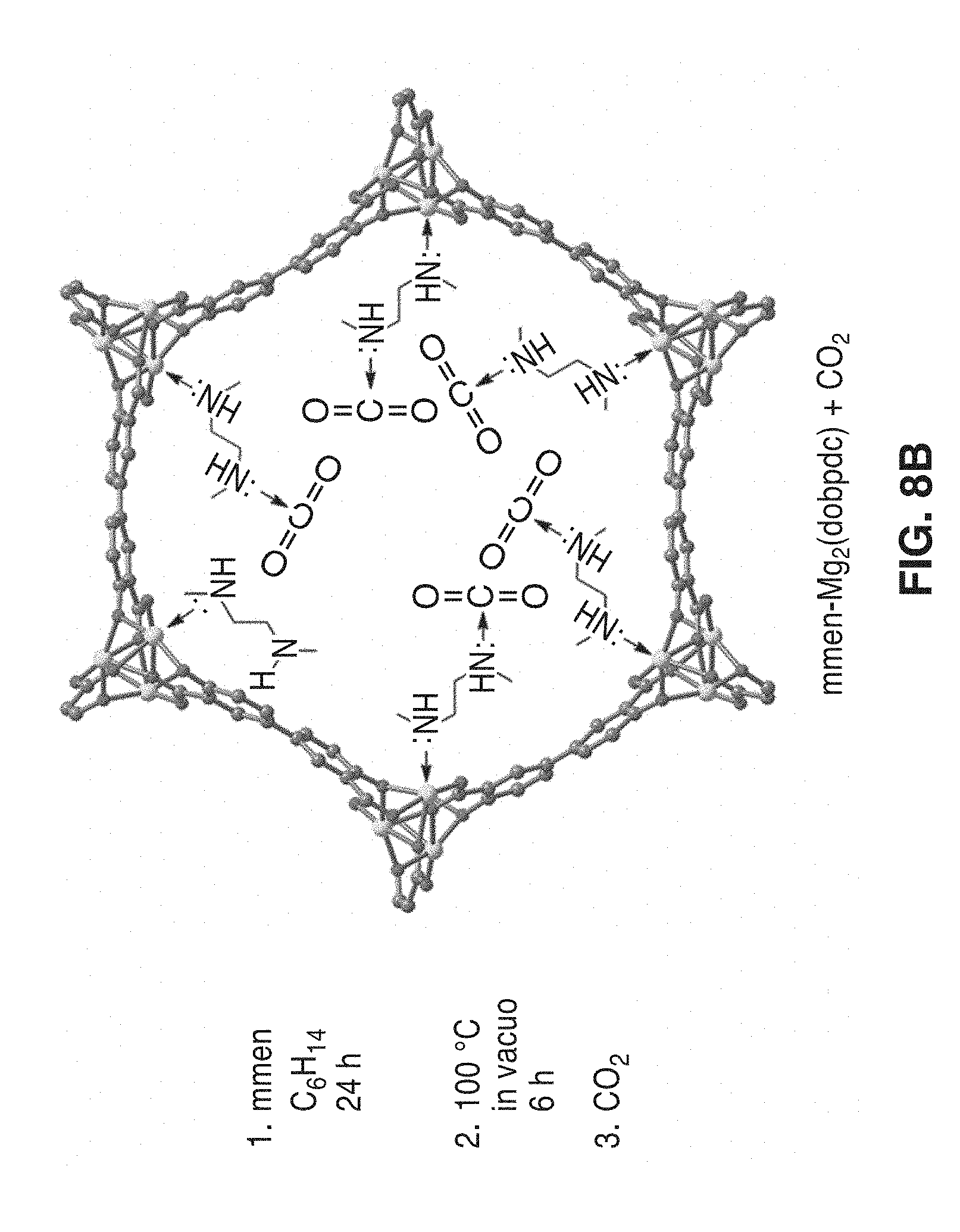

United States Patent
Application |
20190291074 |
Kind Code |
A1 |
Long; Jeffrey R. ; et
al. |
September 26, 2019 |
ALKYLAMINE FUNCTIONALIZED METAL-ORGANIC FRAMEWORKS FOR COMPOSITE
GAS SEPARATIONS
Abstract
Functionalized metal-organic framework adsorbents with ligands
containing basic nitrogen groups such as alkylamines and
alkyldiamines appended to the metal centers and method of isolating
carbon dioxide from a stream of combined gases and carbon dioxide
partial pressures below approximately 1 and 1000 mbar. The
adsorption material has an isosteric heat of carbon dioxide
adsorption of greater than -60 kJ/mol at zero coverage using a
dual-site Langmuir model.
Inventors: |
Long; Jeffrey R.; (Oakland,
CA) ; McDonald; Thomas M.; (Berkeley, CA) ;
D'Alessandro; Deanna M.; (Sydney, AU) |
|
Applicant: |
Name |
City |
State |
Country |
Type |
THE REGENTS OF THE UNIVERSITY OF CALIFORNIA |
Oakland |
CA |
US |
|
|
Assignee: |
THE REGENTS OF THE UNIVERSITY OF
CALIFORNIA
Oakland
CA
|
Family ID: |
48141364 |
Appl. No.: |
16/200988 |
Filed: |
November 27, 2018 |
Related U.S. Patent Documents
|
|
|
|
|
|
Application
Number |
Filing Date |
Patent Number |
|
|
15820350 |
Nov 21, 2017 |
10137430 |
|
|
16200988 |
|
|
|
|
15373426 |
Dec 8, 2016 |
9861953 |
|
|
15820350 |
|
|
|
|
14228532 |
Mar 28, 2014 |
|
|
|
15373426 |
|
|
|
|
PCT/US2012/060915 |
Oct 18, 2012 |
|
|
|
14228532 |
|
|
|
|
61548676 |
Oct 18, 2011 |
|
|
|
Current U.S.
Class: |
1/1 |
Current CPC
Class: |
Y02A 50/20 20180101;
B01D 2258/0283 20130101; Y02C 10/04 20130101; B01J 20/226 20130101;
B01D 53/62 20130101; B01J 20/28057 20130101; Y02C 10/08 20130101;
B01J 20/3425 20130101; Y02C 20/40 20200801; B01D 2253/204 20130101;
B01D 53/02 20130101; C07F 1/08 20130101; B01D 2257/504 20130101;
B01J 20/3483 20130101 |
International
Class: |
B01J 20/22 20060101
B01J020/22; B01D 53/62 20060101 B01D053/62; B01J 20/34 20060101
B01J020/34; B01D 53/02 20060101 B01D053/02; B01J 20/28 20060101
B01J020/28; C07F 1/08 20060101 C07F001/08 |
Goverment Interests
STATEMENT REGARDING FEDERALLY SPONSORED RESEARCH OR DEVELOPMENT
[0003] This invention was made with Government support under
DE-SC0001015 awarded by the Department of Energy. The Government
has certain rights in the invention.
Claims
1. An adsorption material, comprising: a porous metal-organic
framework; and a plurality of ligands within the pores of the
metal-organic framework, each ligand having at least one basic
nitrogen group; wherein the basic nitrogen groups are configured to
selectively adsorb CO.sub.2 from a stream of mixed gases at
pressures below 3 bar and CO.sub.2 partial pressures between 1 and
1000 mbar.
2. A method of separating a mixture stream comprising CO.sub.2 and
N.sub.2, the method comprising: contacting the mixture stream
comprising CO.sub.2 and N.sub.2 with a material comprising a
metal-organic framework, and a ligand with a basic nitrogen group;
wherein the material has an isosteric heat of CO.sub.2 adsorption
of greater than -60 kJ/mol at zero coverage using a dual-site
Langmuir model; obtaining a stream richer in CO.sub.2 as compared
to the mixture stream; and obtaining a stream richer in N.sub.2 as
compared to the mixture stream.
3. A method of separating a mixture stream comprising CO.sub.2 and
other combustion gases, the method comprising: contacting the
mixture stream comprising CO.sub.2 and N.sub.2 with a material
comprising a metal-organic framework and a plurality of ligands
that have at least one basic nitrogen group; obtaining a stream
richer in CO.sub.2 as compared to the mixture stream; and obtaining
a stream richer in N.sub.2 as compared to the mixture stream.
Description
CROSS-REFERENCE TO RELATED APPLICATIONS
[0001] This application is a continuation of U.S. patent
application Ser. No. 15/820,350 filed on Nov. 21, 2017,
incorporated herein by reference in its entirety, now U.S. Pat. No.
10,137,430, incorporated herein by reference in its entirety, which
is a division of U.S. patent application Ser. No. 15/373,426 filed
on Dec. 8, 2016, incorporated herein by reference in its entirety,
now U.S. Pat. No. 9,861,953, incorporated herein by reference in
its entirety, which is a continuation of U.S. patent application
Ser. No. 14/228,532 filed on Mar. 28, 2014, incorporated herein by
reference in its entirety, which is a 35 U.S.C. .sctn. 111(a)
continuation of PCT international application number
PCT/US2012/060915 filed on Oct. 18, 2012, incorporated herein by
reference in its entirety, which claims priority to and the benefit
of U.S. provisional patent application Ser. No. 61/548,676 filed on
Oct. 18, 2011, incorporated herein by reference in its entirety.
Priority is claimed to each of the foregoing applications.
[0002] The above-referenced PCT international application was
published as PCT International Publication No. WO 2013/059527 on
Apr. 25, 2013, incorporated herein by reference in its
entirety.
BACKGROUND OF THE INVENTION
1. Field of Invention
[0004] This invention pertains to the use of metal-organic
frameworks as adsorbents for the separation of composite gasses,
and more particularly to adsorbents with a high concentration of
alkylamine functionalized sites in a metal organic framework and
methods for the separation of a variety of materials based on
selective, reversible electron transfer reactions. For example,
methods are provided for the separation of individual gases from as
stream of combined gases such as CO.sub.2 from N.sub.2 gases or
CO.sub.2 from H.sub.2 gases from a stream of combined gases.
2. Background
[0005] There is a continuing need for the efficient separation of
gas mixtures into their component parts in many different
industrial processes including energy production and emission
reduction. Many gas separations are presently performed on large
scales in numerous industrial processes, often at significant
cost.
[0006] For example, the production of syngas from the conversion of
fossil fuels (natural gas, coal, oil, oil shale, etc) or biomass
requires the separation of CO.sub.2 from H.sub.2 and other useful
gasses. In this context, the coal or other material is converted
into syngas (CO and H.sub.2) which subsequently undergoes the
water-gas shift reaction to generate CO.sub.2 and H.sub.2. The
hydrogen is used to generate electricity after it is separated from
CO.sub.2, which can then be prevented from release into the
atmosphere. This strategy, called pre-combustion CO.sub.2 capture,
is advantageous in comparison to other CO.sub.2 capture
technologies that require separation of CO.sub.2 from N.sub.2,
O.sub.2, or CH.sub.4 because the differences in size and
polarizability between CO.sub.2 and H.sub.2 can be exploited.
Separation of CO.sub.2 from CH.sub.4 is also relevant to the
purification of natural gas, which can have impurity levels of up
to 92% CO.sub.2 at its source. Carbon dioxide removal is required
for approximately 25% of the natural gas reserves in the United
States. Removal of CO.sub.2, which is most commonly accomplished
using amines to reduce CO.sub.2 levels to the required 2% maximum,
is conducted at pressures between 20 bar and 70 bar. Carbon dioxide
removal is required for approximately 25% of the natural gas
reserves in the United States.
[0007] Gas separations are also important in post-combustion of
fossil fuels for energy production. The combustion of fossil fuels
is largely responsible for the increase in the global concentration
of CO.sub.2 in the Earth's atmosphere, yet fossil fuels will
continue to be heavily utilized for energy production during the
21st century.
[0008] The development of more efficient processes for capturing
CO.sub.2 from power plant flue streams is critical for the
reduction of greenhouse gas emissions implicated in global warming.
Currently, there is significant interest in the development and
implementation of technologies that slow CO.sub.2 emissions and
thus forestall the most severe consequences of global warming. For
limiting future CO.sub.2 emissions from large, stationary sources
like coal-fired power plants, carbon capture and sequestration
(CCS) has been proposed. The CCS process involves the selective
removal of CO.sub.2 from gas mixtures, the compression of pure
CO.sub.2 to a supercritical fluid, transportation to an injection
site, and finally permanent subterranean or submarine storage. For
the retrofit of existing power plants, post-combustion CO.sub.2
capture is a likely configuration. In this design, fuel is burned
in air and CO.sub.2 is removed from the effluent. For coal-fired
power plants, the largest flue gas components by volume are N.sub.2
(70-75%), CO.sub.2 (15-16%), H.sub.2O (5-7%) and 02 (3-4%), with
total pressures near 1 bar and temperatures between 40.degree. C.
and 60.degree. C. For post-combustion CO.sub.2 capture, maximizing
adsorption capacity for CO.sub.2 at low pressures is highly
desirable. Because the partial pressure of CO.sub.2 in flue gas
emitted from coal fired power stations is typically between 0.10
and 0.15 bar, the simplest approximation for the capacity of
materials being considered is the quantity of gas adsorbed at these
lower pressures, not the capacity at 1 bar.
[0009] Aqueous amine solutions are currently the most viable
adsorbents for carbon capture and are presently used for the
removal of CO.sub.2 from industrial commodities like natural gas.
While a variety of advanced amines are available, 30%
monoethanolamine (MEA) in water is the benchmark solvent against
which competing technologies are generally compared. The low
solvent cost and proven effectiveness make MEA an attractive
adsorbent for many applications.
[0010] Conventional CO.sub.2 capture processes involving the
chemisorption of CO.sub.2 by alkylamine-containing liquids present
several disadvantages, including the considerable heat required to
regenerate the liquid, solution boil-off and the necessary use of
inhibitors for corrosion control. Therefore, if MEA were to be
utilized for carbon capture and sequestration, electricity prices
are projected to increase by 86%.
[0011] The formation of ammonium carbamate from two MEA molecules
and one CO.sub.2 molecule endows the scrubber with extremely high
selectivity for CO.sub.2, but significant energy is required to
regenerate the solution. This high regeneration energy cost has two
primary components: first, the strong, chemisorptive bond between
the carbon dioxide and the amine must be broken; second, a large
amount of spectator water solvent must be heated and cooled along
with the active amine adsorbent, giving rise to an inefficient
system. Because amines are corrosive to plant infrastructure,
solutions are typically limited to no more than 30% (w/w) of the
amine, and a significant increase in this concentration is not
deemed feasible. In addition, solvent boil-off occurs during
repeated regeneration cycles consuming the scrubber and increasing
costs. The diversion of steam from the electricity generation cycle
to the solvent regeneration cycle sharply reduces the net
electricity output of the plant, drastically increasing electricity
costs. It has been demonstrated that plant efficiency is highly
dependent on the solvent regeneration energy that is needed. These
limitations represent the most significant obstacles to wider
implementation of amine scrubbing technologies for post-combustion
carbon capture.
[0012] Attempts to address these limitations have focused on the
adsorption of CO.sub.2 in porous solids such as zeolites and
amine-modified silicas via the formation of carbamate or
bicarbonate species. The viability of the materials under realistic
flue stream conditions requires air and water stability, corrosion
resistance, high thermal stability, and high selectivity for
CO.sub.2 over other components in flue gas. Currently, aqueous
amines are used industrially to separate CO.sub.2 from gas mixtures
with high CO.sub.2 partial pressures like natural gas, while some
solid adsorbents are used to remove CO.sub.2 from mixtures with low
CO.sub.2 partial pressures. In addition to the separation of
combustion gases, there are a number of current industrial
processes that utilize liquid or solid adsorbents to remove
CO.sub.2 from gas mixtures.
[0013] Accordingly, there is a need for an efficient methods and
materials for selectively separating constituent gases from a
stream of gases that can be performed at lower temperatures and
pressures than existing techniques. There is also a need for
materials and methods that provide selective, reversible electron
transfer reactions and associated functions such as catalysis,
including oxidation as well as gas storage. The present invention
satisfies these needs as well as others and is generally an
improvement over the art.
SUMMARY OF THE INVENTION
[0014] The present invention is directed to metal-organic framework
materials and methods for use in a variety of gas separation and
manipulation applications including the isolation of individual
gases from a stream of combined gases, such as carbon
dioxide/nitrogen, carbon dioxide/hydrogen, carbon dioxide/methane,
carbon dioxide/oxygen, carbon monoxide/nitrogen, carbon
monoxide/methane, carbon monoxide/hydrogen, hydrogen
sulfide/methane and hydrogen sulfide/nitrogen.
[0015] Among the primary benefits of physiorption onto solid
materials is the low regeneration energy compared to that required
for aqueous amines. However, this benefit frequently comes at the
expense of low capacity and poor selectivity. The present invention
provides adsorbents that bridge the two approaches through the
incorporation of sites that bind CO.sub.2 by chemisorption onto
solid materials. The new materials may eliminate the need for
aqueous solvents, and may have significantly lower regeneration
costs compared with traditional amine scrubbers, yet maintain their
exceptional selectivity and high capacity for CO.sub.2 at low
pressures.
[0016] The adsorption materials for gas separations are
metal-organic frameworks containing ligands with basic nitrogen
groups. Metal-organic frameworks are porous, crystalline solids
that are preferably functionalized with the incorporation of
alkylamines, which exhibit enhanced basicity over aromatic amines
and are capable of strongly adsorbing acid gases.
[0017] The preferred metal-organic frameworks are a group of porous
crystalline materials formed of metal cations or clusters joined by
multitopic organic linkers. By way of example, and not of
limitation, the invention provides functional materials made from
metal-organic framework adsorbents with a framework selected from
the group: M-BTT (M=Ca, Fe, Mn, Cu, Co, Ni, Cr, Cd); M-BTTri (M=Cr,
Mn, Fe, Co, Ni, Cu); M-BTP (M=Co, Ni, Zn); M.sub.3(BTC).sub.2
(M=Cu, Cr); M.sub.2(dobdc) (M=Mg, Ca, Mn, Cr, Fe, Co, Ni, Cu, Zn);
M.sub.2(dobpdc) (M=Mg, Ca, Mn, Cr, Fe, Co, Ni, Cu, Zn), and MIL-100
(M=Fe, Al, Cr, Ti, Sc, V); MIL-101 (M=Fe, Al, Cr, Ti, Sc, V). The
metal-organic framework may also include open metal sites.
[0018] Ligands of the metal-organic framework may contain other
structural elements used to coordinate the ligand to one or more
metals of the framework. These include but are not limited to the
following functional groups: carboxylate, triazolate, pyrazolate,
tetrazolate, pyridines, amines, alkoxide and/or sulfate groups. The
preferred alkylamine ligand is N,N'-dimethylethylenediamine
("mmen") producing (mmen-Mg.sub.2-BTTri) or mmen-Mg.sub.2.(dopbdc)
functionalized frameworks.
[0019] The removal of dilute concentrations of acid gases by highly
selective adsorption, as demonstrated by the affinity of
(mmen-CuBTTri) for CO.sub.2 versus N.sub.2, is used to illustrate
the use of the adsorbents in post-combustion CO.sub.2 capture
applications as well as other gas separation applications.
[0020] The basic nitrogen groups may be incorporated into the
framework on a ligand prior to framework formation, through
substitution or modification of a functional group that was bonded
to a ligand prior to framework formation, or by substitution of a
ligand after framework formation with the ligand with a basic
nitrogen group.
[0021] Due to their high surface areas and low bulk densities,
these materials demonstrate remarkable working capacities for
sequestering carbon dioxide, making them ideal for use in large
scale processing plants and a great improvement over current
adsorbents. The successful implementation of these new adsorbents
could reduce the substantial energy cost of adsorbing CO.sub.2
emissions resulting from the combustion of fossil fuels, including
coal and natural gas.
[0022] Another embodiment is a method of separating a mixture
stream comprising CO.sub.2 and N.sub.2. The method includes
contacting the mixture stream including CO.sub.2 and N.sub.2 with a
material comprising a metal-organic framework, and a ligand with a
basic nitrogen group, wherein the material preferably has an
isosteric heat of CO.sub.2 adsorption of greater than -70 kJ/mol at
zero coverage as determined by the Clausius-Clapeyron relation,
obtaining a stream richer in CO.sub.2 as compared to the mixture
stream, and obtaining a stream richer in N.sub.2 as compared to the
mixture stream.
[0023] According to one aspect of the invention, a process is
provided for attaching polyamine ligands to the surface of
metal-organic frameworks with exposed metal cations for use in
CO.sub.2 capture.
[0024] Another aspect of the invention is to provide a porous
adsorbent material with a metal organic framework functionalized
with N,N'-dimethylethylenediamine ("mmen") with frameworks of the
family M-BTTri where M=(Cr, Mn, Fe, Co, Ni or Cu) with
H.sub.3[(Cu.sub.4Cl).sub.3(BTTri).sub.8 (mmen).sub.12] particularly
preferred for the separation of gases from a mixture of gases at
low pressures below approximately 1 bar.
[0025] According to another aspect of the invention, a
functionalized metal organic framework is provided that can
separate gases at low temperatures and pressures.
[0026] Yet another aspect of the invention is to provide a material
and method for pre-combustion separation of carbon dioxide and
hydrogen and methane from a stream of gases.
[0027] A further aspect of the invention is to provide a material
and method for separation of carbon dioxide from a stream of
post-combustion flue gases at low pressures and concentrations.
[0028] Another aspect of the invention is to provide a
metal-organic framework that is adaptable to many different
separation needs.
[0029] Further aspects of the invention will be brought out in the
following portions of the specification, wherein the detailed
description is for the purpose of fully disclosing preferred
embodiments of the invention without placing limitations
thereon.
BRIEF DESCRIPTION OF THE DRAWINGS
[0030] The invention will be more fully understood by reference to
the following drawings which are for illustrative purposes
only:
[0031] FIG. 1 is a representation of a portion of the structure of
the amine functionalized metal-organic framework mmen-CuBTTri, with
incorporation of the diamine N,N'-dimethylethylenediamine onto open
metal sites within the pores according to the invention.
[0032] FIG. 2A is a graph plotting gravimetric gas sorption
isotherms for CO.sub.2 (squares) and N.sub.2 (circles) adsorption
at 25.degree. C. for mmen-CuBTTri and CuBTTri. The horizontal
dashed line in corresponds to 10 wt % CO.sub.2 adsorption.
[0033] FIG. 2B is a graph plotting volumetric gas sorption
isotherms for CO.sub.2 (squares) and N.sub.2 (circles) adsorption
at 25.degree. C. for mmen-CuBTTri and CuBTTri for comparison to
FIG. 2A.
[0034] FIG. 3 is a graph of infrared spectra obtained upon exposure
of mmen-CuBTTri to a 5% CO.sub.2/95% He gas mixture in a
high-pressure DRIFTS cell. Under dry conditions, the N--H stretch
of mmen is apparent at 3283 cm.sup.-1 (vertical dashed line) on a
fully evacuated sample (28). Dilute CO.sub.2 in He was slowly
introduced into the cell (30) up to a dynamic pressure of 1.5 bar
(32). Upon saturation, the N--H stretch fully disappeared.
Following reactivation under vacuum and heating at 60.degree. C.
(34), the N--H stretch reappeared.
[0035] FIG. 4 is a graph of CO.sub.2 adsorption isotherms at 298 K
(squares), 308 K (circles) and 318 K (triangles) for
mmen-CuBTTri.
[0036] FIG. 5 is a graph of N.sub.2 adsorption isotherms at 298 K
(squares), 308 K (circles) and 318 K (triangles) for
mmen-CuBTTri.
[0037] FIG. 6 is a graph of Isosteric heats of adsorption for
mmen-CuBTTri calculated from the viral method (circles) and the
dual-site Langmuir method (squares).
[0038] FIG. 7 is a graph of % mass change over time with repeated
adsorption cycles. Upon introduction of a 15% CO.sub.2 mixture in
N.sub.2 at 25.degree. C., the mass of mmen-CuBTTri increased by
nearly 7% as measured by thermogravimetric analysis. Upon
saturation, a N.sub.2 purge flow with a temperature swing to
60.degree. C. fully regenerated the material, with no apparent
capacity loss after 72 cycles.
[0039] FIG. 8A and FIG. 8B depict a synthesis scheme for
mmen-Mg.sub.2(dobpdc) where (mmen=N,N'-dimethylethylenediamine) and
(dobpdc.sup.4-=4,4'-dioxido-3,3'-biphenyldicarboxylate). From the
microwave reaction of MgBr.sub.2.6H.sub.2O and H.sub.4dobpdc,
Mg.sub.2(dobpdc) is obtained following evacuation of the as
synthesized solid at high temperatures (middle). Addition of an
excess of mmen to the evacuated framework yields the amine-appended
CO.sub.2 adsorbent
Mg.sub.2(dobpdc)(mmen).sub.1.6(H.sub.2O).sub.0.4.
[0040] FIG. 9 is a graph of the adsorption of CO.sub.2 in
mmen-Mg.sub.2-(dobpdc) at 25.degree. C. (squares), 50.degree. C.
(triangles), and 75.degree. C. (circles). Inset: The isotherms at
very low pressures exhibit a step that shifts to higher pressures
at higher temperatures. The dashed, vertical line marks the current
partial pressure of CO.sub.2 in air (390 ppm).
DETAILED DESCRIPTION OF THE INVENTION
[0041] Referring more specifically to the drawings, for
illustrative purposes several embodiments of the metal-organic
framework adsorbents of the present invention are depicted
generally in FIG. 1 through FIG. 9 and the associated methods for
using and producing the alkylamine functionalized frameworks. It
will be appreciated that the methods may vary as to the specific
steps and sequence and the metal-organic framework architecture may
vary as to structural details, without departing from the basic
concepts as disclosed herein. The method steps are merely exemplary
of the order that these steps may occur. The steps may occur in any
order that is desired, such that it still performs the goals of the
claimed invention.
[0042] Metal-organic frameworks are a class of porous, crystalline
adsorbents that enables greater functionality with reduced
adsorbent mass and volume compared to traditional solid adsorbents.
These metal-organic frameworks are preferred because of the
presence of coordinatively unsaturated metal centers (open metal
sites) along the pore surfaces. These coordinate metal cations are
known to behave as Lewis acids that strongly polarize gas
adsorbents and are further amenable to post-synthetic
functionalization. In these frameworks with well separated open
metal sites, one amine of a diamine ligand molecule can bind to a
metal cation as a Lewis base while the second amine remains
available as a chemically reactive adsorption site. The metals in
the framework can be individual metal atoms bridged by a set of
ligands or metal clusters (a collection of metal atoms that as a
group interact with a set of ligands.
[0043] The preferred metal-organic frameworks are a group of porous
crystalline materials formed of metal cations or clusters joined by
multitopic organic linkers. These are frequently frameworks that
are described as having "open metal sites" (also called
coordinatively unsaturated metal centers).
[0044] By way of example, and not of limitation, the invention
provides functional materials made from metal-organic framework
adsorbents selected from the group: M-(1,3,5-benzenetristriazolate)
where (M=Cr, Mn, Fe, Co, Ni or Cu). Other basic framework examples
that can be functionalized with alkylamines and used for low
pressure applications include: M-BTT (M=Ca, Fe, Mn, Cu, Co, Ni, Cr,
Cd) (BTT=1,3,5-benzenetristetrazolate); M-BTP where (M=Co, Ni, Zn)
and (BTP=1,3,5-benzenetrispyrazolate); M.sub.3(BTC).sub.2 where
(M=Cu, Cr) and (BTC=1,3,5-benzenetriscarboxylate); M.sub.2(dobdc)
where (M=Mg, Ca, Mn, Cr, Fe, Co, Ni, Cu, Zn) and
(dobdc=2,5-dioxido-1,4-benzenedicarboxylate); M.sub.2(dobpdc) where
(M=Mg, Ca, Mn, Cr, Fe, Co, Ni, Cu, Zn) and
(dobpdc=4,4'-dioxido-3,3'-biphenyldicarboxylate); MIL-100 where
(M=Fe, Al, Cr, Ti, Sc, V) and
(Ligand=BTC=1,3,5-benzenetriscarboxylate); and MIL-101 where (M=Fe,
Al, Cr, Ti, Sc, V) and (Ligand=BDC=1,4-benzenedicarboxylate).
[0045] The ligands of the metal-organic frameworks preferably
contain basic nitrogen groups. These basic nitrogen ligands may
include, for example, alkyl amines or imines, but not aromatic
amines (i.e. aniline derivatives).
[0046] Some or all ligands of the metal-organic framework include
functional groups that are not coordinated to metal cations and are
available to form reversible weak chemical bonds with CO.sub.2.
Preferably, the reactive chemical atom contains a lone pair of
electrons including nitrogen, oxygen, sulfur, and phosphorous. More
preferably, it is a basic amine. More preferably, the lone pair or
pairs of the reactive atom are not in resonance with an aromatic
ring. Most preferably, the functional group is a primary,
secondary, or tertiary alkylamine (an aliphatic amine).
[0047] Ligands of the metal-organic framework may contain other
structural elements used to coordinate the ligand to one or more
metals of the framework. These include but are not limited to the
following functional groups: carboxylate, triazolate, pyrazolate,
tetrazolate, pyridines, amines, alkoxide and/or sulfate groups. The
preferred alkylamine ligand is N,N'-dimethylethylenediamine
("mmen") producing (mmen-Mg.sub.2-BTTri) or mmen-Mg.sub.2.(dopbdc)
functionalized frameworks.
[0048] Some or all ligands of the metal organic framework may
contain one or more aromatic rings that contain carbon and may
contain other atoms including boron, nitrogen, and oxygen. This is
most preferably five and six membered rings. These rings may
provide structural rigidity to the material and/or provide spatial
separation of other functional groups contained within ligands as
to provide porosity to the adsorbent.
[0049] The structure of the basic ligands may include three
distinct components: 1) A backbone that provides structural
rigidity to the material. This may be, for example, an aromatic
group such as a phenyl group. 2) At least one functional group that
binds the ligands to the metal such as nitrogen or oxygen atoms.
Specific examples include a carboxylate group, a triazolate group
(as in this case for Cu-BTTri), pyrazolates, tetrazolates,
pyridines, and sulfates. 3) A functional group that contains a
nitrogen atom that is not integral to the structural rigidity of
the material and is not bound to a metal that is available to
interact with gases.
[0050] The functional group that contains a nitrogen atom and
interacts with the CO.sub.2 molecule is preferably a basic organic
group. Preferred functional groups include primary amines,
secondary amines, tertiary amines, primary imines, and secondary
imines, and similar compounds. Although these groups all contain
nitrogen, in alternative embodiments, these groups could include
other atoms as well, especially atoms having an available lone pair
of electrons.
[0051] These basic nitrogen functional groups can be incorporated
into the metal-organic framework in one of three preferable ways:
1) attached as a functional group on a ligand prior to framework
formation; 2) as a substitution or modification of a functional
group that was bonded to a ligand prior to framework formation; and
3) as a substitution of a ligand after framework formation for a
new ligand that contains the desired functional group.
[0052] If the amines are attached to the ligand prior to framework
formation as in method 1) described above, for incorporating a
basic nitrogen group into the framework, the nitrogen is preferably
not directly bonded to an aromatic carbon atom. This is because the
nitrogen would be bonded to an alkylcarbon (a methylene), giving
rise to an alkylamine groups, which is preferred. Accordingly, it
is preferred that at least one atom of any type separates the amine
that interacts with CO.sub.2 from the aromatic backbone.
[0053] In method 2), the basic nitrogen groups are incorporated
into the framework through a substitution or modification of a
functional group that was bonded to a ligand prior to framework
formation and the ligands are not exchanged. Rather, functional
groups within the ligands may be exchanged for the desired
functionality. This could potentially include modification of C--H
bonds by aromatic substitution reactions, nucleophilic substitution
reactions (including replacement of alkyl halide groups for alkyl
amine groups), condensation reactions (including conversions of
aldehydes to imines), and reductions (including imines to alkyl
amines, nitriles to alkylamines, and amides to alkyl amines). Other
potential reactions could also be used that modify ligands to
include alkylamines or imines after framework synthesis.
[0054] Finally, with method 3) for incorporating a basic nitrogen
group into the framework, the metal ligands are exchanged. The new
ligand preferably has at least two functional groups: 1) A
functional group used to bind CO.sub.2 and 2) a functional group
used to bind to the metal. The second functional group that binds
the metal can also be an amine. It is possible to use other
functional groups such as oxygen containing groups like alcohols,
ethers or alkoxides, carbon groups like carbenes or unsaturated
bonds like alkenes or alkynes, or sulfur atoms.
[0055] Preferable characteristic for the end that binds the metal
include the following: 1) strongly bonded to the metal so the
functional groups are not removed upon framework activation by
vacuum; 2) capable of being grafted at nearly all metal sites
within the pores for nearly complete functionalization. The ligand
itself may contain one or more amines that bind CO.sub.2. For
example, the ligand could have 3 carboxylate groups for binding
metals and 1, 2, or 3 (or more) alkylamine groups on each ligand.
Examples may include Tris(2-aminoethyl)amine (primary and tertiary
amines) or Tris[2(methylamino)ethyl]amine (secondary and tertiary
amines), which would be capable of binding a metal-site with one
amine and adsorbing CO.sub.2 with 3 other amines. These examples
are branched.
[0056] Other alternatives are linear like tetraethylenepentamine (2
primary amines and 3 tertiary amines) or Diethylenetriamine (2
primary amines and 1 secondary amine). In the case of mmen-CuBTTri,
described herein, it is believed that each amine binds one
CO.sub.2. However, it is also possible for two or more amines to
bind a single CO.sub.2, especially in ligands with multiple
amines.
[0057] Amines do not necessarily simply polarize CO.sub.2; rather,
they strongly and selectively bind it through chemisorptive
interactions. Amines tethered to solid surfaces within porous
materials also have considerable advantages over aqueous
alkanolamines. It has also been found that the incorporation of
alkylamine groups at higher loadings can further polarize the
overall surface area of a metal-organic framework, thereby
increasing the capacity for CO.sub.2 capture. Other functional
groups are similarly capable of polarizing framework surfaces, but
many are not capable of undergoing the chemisorptive type process.
Higher order amines, in particular secondary amines, have more
favorable adsorption characteristics in solutions as well as on
solid adsorbents. It has been found that the incorporation of
N,N'-dimethylethylenediamine (mmen) at high loadings within CuBTTri
affords a material with exceptional CO.sub.2 capture
characteristics.
[0058] Generally, a method for separating constituent gases from
stream of mixed gases containing at least a first gas and a second
gas is provided with the use of an adsorbent of a metal-organic
framework adsorbent of the group M-BTT (M=Ca, Fe, Mn, Cu, Co, Ni,
Cr, Cd); M-BTTri (M=Cr, Mn, Fe, Co, Ni, Cu); M-BTP (M=Co, Ni, Zn);
M.sub.3(BTC).sub.2 (M=Cu, Cr); M.sub.2(dobdc) (M=Mg, Ca, Mn, Cr,
Fe, Co, Ni, Cu, Zn); M.sub.2(dobpdc) (M=Mg, Ca, Mn, Cr, Fe, Co, Ni,
Cu, Zn); MIL-100 (M=Fe, Al, Cr, Ti, Sc, V) or MIL-101 (M=Fe, Al,
Cr, Ti, Sc, V) that have been functionalized with ligands
containing basic nitrogen groups including but not limited to
alkyldiamine ligands. The stream of mixed gases is directed across
a bed of adsorbent and the molecules of the first gas are adsorbed
onto the metal-organic framework so that the resulting stream is
richer in the second gas as compared to the mixture stream that is
collected. The adsorbed first gas is released from the
metal-organic framework to obtain a stream richer in the first
chemical as compared to the mixture stream that is also collected.
The adsorbed chemical is typically released by a change in
temperature or pressure. A purge gas may also be used to move the
released gas through the bed for collection.
[0059] It will be seen that the selection of the metal cations and
organic framework structure can be tailored by the type of gases to
be separated and the temperature and pressure conditions of the
separation. M.sub.2(dobpdc) and CuBTTri functionalized with
alkylamines are frameworks that are particularly suited for carbon
dioxide/nitrogen gas separations at CO.sub.2 partial pressures
between 1 and 1000 mbar.
[0060] Two frameworks, mmen-CuBTTri shown in FIG. 1 and
mmen-Mg.sub.2(dobpdc) shown in FIG. 8B, are used to illustrate the
M-BTTri and M.sub.2(dobpdc) framework families and the methods of
use for gas separations.
[0061] Turning now to FIG. 1, an embodiment of a portion of a
metal-organic framework crystal structure of a mmen-CuBTTri, a
water stable, triazolate-bridged framework of the invention is
schematically shown. The incorporation of the
N,N'-dimethylethylenediamine (mmen) ligand into the (CuBTTri)
framework, was shown to drastically enhance CO.sub.2 adsorption.
The attachment of the mmen alkylamine at the metal centers of the
framework is shown with arrows. Because the diamines are shorter
than the distance between two adjacent metal sites one amine from
each molecule is bound to a single metal site, while the other
amine is free to interact with guest gas molecules upon framework
activation.
[0062] High porosity is maintained despite stoichiometric
attachment of mmen to the open metal sites of the framework,
resulting in a BET surface area of 870 m.sup.2/g. At 25.degree. C.
under a 0.15 bar CO.sub.2/0.75 bar N.sub.2 mixture, mmen-CuBTTri
adsorbs 2.38 mmol CO.sub.2/g (9.5 wt %) with a selectivity of 327,
as determined using Ideal Adsorbed Solution Theory (IAST). The high
capacity and selectivity are consequences of the exceptionally
large isosteric heat of CO.sub.2 adsorption, calculated to be -96
kJ/mol at zero coverage. Infrared spectra support chemisorptions
between amines and CO.sub.2 as one of the primary mechanisms of
uptake. Despite the large initial heat of adsorption, the CO.sub.2
uptake was fully reversible and the framework could be easily
regenerated at 60.degree. C., enabling a cycling time of just 27
minutes with no loss of capacity over the course of 72
adsorption/desorption cycles.
[0063] Overall, the performance characteristics of CuBTTri indicate
it to be an exceptional new adsorbent for CO.sub.2 capture,
comparing favorably with both amine-grafted silicas and aqueous
amine methods.
[0064] The large capacity, high selectivity, and fast kinetics of
these materials for adsorbing CO.sub.2 from dry gas mixtures with
N.sub.2 and O.sub.2 make the functionalized metal-organic
frameworks attractive new adsorbents for applications in which
zeolites and liquid adsorbents are currently used, including the
removal of CO.sub.2 from flue gases at low pressures below
approximately 1 bar.
[0065] The invention may be better understood with reference to the
accompanying examples, which are intended for purposes of
illustration only and should not be construed as in any sense
limiting the scope of the present invention as defined in the
claims appended hereto.
Example 1
[0066] In order to demonstrate the functionality of metal-organic
frameworks featuring coordinatively-unsaturated metal centers for
separating gases, a mmen-CuBTTri framework as shown in FIG. 1 was
constructed and tested.
[0067] A sample of CuBTTri (100.0 mg, 32.4/.mu.mol) was suspended
in 10 mL of anhydrous hexane under nitrogen, and 75.4 .mu.L (61.2
.mu.g, 701 .mu.mol, 1.8 equivalents per unsaturated CU.sup.II site)
of N,N' dimethylethylenediamine (mmen) was added via micropipette
with stirring. The compound immediately turned blue and the
suspension was heated at reflux for 18 hours under nitrogen. The
solid was collected by filtration and washed with successive
aliquots of hexane (5.times.10 mL) to remove unreacted diamine. The
solid was then dried under reduced pressure to remove hexane. Anal.
Calcd. for C.sub.144H.sub.195Cl.sub.3Cu.sub.12N.sub.96 (Mw=4139.6
g/mol): C, 41.78, H, 4.75, N, 32.48. Found: C, 42.23, H, 4.47, N,
32.05.
[0068] The grafted material, mmen-CuBTTri, was then activated by
heating at 50.degree. C. for 24 hours under a dynamic vacuum prior
to gas adsorption. Nitrogen adsorption isotherms collected at 77 K
indicate a BET surface area of 870 m.sup.2/g, while powder x-ray
diffraction data show the structure of the CuBTTri framework to be
intact. Overall, the characterization data are most consistent with
a chemical formula of
H.sub.3[(CU.sub.4Cl).sub.3(BTTri).sub.8(mmen).sub.12], with
approximately one mmen molecule for each available metal site.
Thus, mmen-CuBTTri is thought to possess a high concentration of
surface-appended mmen molecules, where one of the amine groups is
bound to a Cu.sup.2+ center, while the other dangles within the
pore, as depicted in FIG. 1.
[0069] Using the same procedure, another framework:
H.sub.3[(Cu.sub.4Cl).sub.3(BTTri).sub.8(men).sub.6].2H.sub.2O
(men-CuBTTri) was produced and evaluated for comparison. 1.8
equivalents of N-methylethylenediamine (men) was added to activated
CuBTTri in hexane, giving rise a blue material. Anal. Calcd. for
C.sub.114H.sub.115C.sub.13CU.sub.12N.sub.84O.sub.2 (Mw=3562.6
g/mol): C, 38.43, H, 3.25, N, 33.02. Found: C, 38.55, H, 3.23, N,
32.51.
Example 2
[0070] In order to demonstrate the functionality of metal-organic
frameworks featuring coordinatively-unsaturated metal centers for
separating gases, a mmen-CuBTTri framework as shown in FIG. 1 was
constructed and tested.
[0071] The mmen-CuBTTri and men-CuBTTri adsorbents were initially
tested in the context of separating a mixture stream including
CO.sub.2 and N.sub.2 to obtain a stream richer in N.sub.2 as
compared to the mixture stream, with the adsorption of CO.sub.2 in
the frameworks at low pressures.
[0072] Gas adsorption isotherms for pressures in the range 0-1.1
bar were measured by a volumetric method using a Micromeritics
ASAP2020 instrument. A sample was transferred in an N.sub.2 filled
glovebag to a pre-weighed analysis tube, which was capped with a
transeal and evacuated by heating (50.degree. C. for mmen-CuBTTri,
100.degree. C. for men-CuBTTri) under dynamic vacuum for 24 hours.
The evacuated analysis tube containing the degassed sample was then
carefully transferred to an electronic balance and weighed again to
determine the mass of sample (108.5 mg for mmen-, 69.2 mg for
men-CuBTTri). The tube was then transferred back to the analysis
port of the gas adsorption instrument. For all isotherms, warm and
cold free space correction measurements were performed using
ultra-high purity Helium gas (UHP grade 5.0, 99.999% purity);
N.sub.2 isotherms at 77 K were measured in liquid nitrogen using
UHP-grade gas sources. CO.sub.2 and N.sub.2 isotherms at 298, 308
and 318 K were measured using a Julabo isothermal bath with
UHP-grade gases. Oil-free vacuum pumps and oil-free pressure
regulators were used for all measurements to prevent contamination
of the samples during the evacuation process or of the feed gases
during the isotherm measurements.
[0073] The incorporation of mmen in the CuBTTri framework resulted
in a material with excellent CO.sub.2 adsorption characteristics.
As shown in FIG. 2A and FIG. 2B, mmen-CuBTTri displays
significantly enhanced CO.sub.2 adsorption at all pressures between
0 and 1.1 bar relative to the unappended framework.
[0074] Thermogravimetric analyses were carried out at a ramp rate
of 1.degree. C./min under a nitrogen flow with a TA Instruments TGA
Q5000 V3.1 Build 246. CO.sub.2 cycling experiments were performed
using 15% CO.sub.2/N.sub.2 (Praxair Certified standard NI-CD15C-K)
and N.sub.2 (Praxair, 99.99%). A flow rate of 25 mL/min was
employed for both gases. Prior to cycling, the sample was activated
by heating at 60.degree. C. for 1 hour, followed by cooling to
25.degree. C. under an N.sub.2 atmosphere. Sample mass was
normalized to be 0% at 25.degree. C. under an N.sub.2 atmosphere.
Masses were uncorrected for buoyancy effects, which were small
compared to mass changes realized from gas adsorption.
[0075] The crystallite volumetric capacities of CuBTTri and
mmen-CuBTTri were determined from unit cell densities. Only slight
changes to the unit cell length were apparent from the PXRD
diffraction patterns upon addition of mmen to the open-metal sites
of CuBTTri. For both samples, a unit cell length of a=18.647 A and
a unit cell volume of V=6483.8 A3 were used for density
calculations. The molecular weight of mmen-CuBTTri was calculated
to have 1 mmen for each open metal site, while CuBTTri was
calculated to have no guest solvent molecules present. Gas sorption
data was converted from mmol/g to mmol/cm.sup.3 with the density of
CuBTTri: p=0.789 g/cm.sup.3 D and the density of mmen-CuBTTri:
p=1.059 g/cm.sup.3
[0076] Quantifying the extent of the improvement in CO.sub.2
adsorption between CuBTTri and mmen-CuBTTri is not trivial, since
the degree of improvement depends significantly on the units to
which the gas uptake is normalized. FIG. 2A plots the gravimetric
gas adsorption isotherm, while FIG. 2B plots the crystallite
volumetric gas adsorption isotherm for the two materials.
[0077] FIG. 2A is a graph plotting gravimetric gas sorption
isotherms for CO.sub.2 (squares) 12 and N.sub.2 (circles) 16
adsorption at 25.degree. C. for mmen-CuBTTri. Gravimetric gas
sorption isotherms for CO.sub.2 (squares) 14 and N.sub.2 (circles)
18 for adsorption at 25.degree. C. for CuBTTri alone is also
plotted for comparison. The horizontal dashed line in corresponds
to 10 wt % CO.sub.2 adsorption.
[0078] FIG. 2B is a graph plotting volumetric gas sorption
isotherms for CO.sub.2 (squares) 20 and N.sub.2 (circles) 24
adsorption at 25.degree. C. for mmen-CuBTTri. Volumetric gas
sorption isotherms for CO.sub.2 (squares) 22 and N.sub.2 (circles)
26 adsorption at 25.degree. C. for CuBTTri alone is also plotted
for comparison for comparison to FIG. 3A.
[0079] The volumetric capacity for an actual adsorber unit is
dependent upon how crystallites pack together and the fraction of
void space within the occupied volume. Yet, gravimetric capacity
alone does not provide a complete measure of the performance of a
material being proposed for stationary applications, such as
post-combustion CO.sub.2 capture. Here, infrastructure costs are
linked more directly to the volume the adsorbent would occupy than
to its mass. Because incorporation of mmen into CuBTTri increases
the framework density by 34% with no significant change in volume,
this system is a good candidate for comparisons between gravimetric
and volumetric capacities.
[0080] It is important to note, however, that no single-crystal
diffraction data are available for either CuBTTri or mmen-CuBTTri.
Framework volumes are based upon powder pattern unit cell
optimizations, and framework compositions are based upon elemental
and thermogravimetric analyses.
[0081] At 25.degree. C. and 1 bar, mmen-CuBTTri adsorbs 4.2 mmol/g
of CO.sub.2 (15.4 wt %), representing a 15% improvement in
gravimetric capacity compared to the unmodified CuBTTri framework.
However, CO.sub.2 comprises at most 15% of coal fired power station
flue gas and the effluent is released into the environment at total
pressures near 1 bar. Thus, the more important criterion for
CO.sub.2 capacity is that of the framework at a pressure near 0.15
bar. At 25.degree. C. and 0.15 bar of CO.sub.2, mmen-CuBTTri
adsorbs 2.38 mmol/g (9.5 wt %). Note that 2.90 mmol/g would
correspond to the adsorption of one CO.sub.2 molecule per mmen in
the functionalized framework. Under the same conditions, the
unmodified framework only adsorbs 0.69 mmol/g (2.9 wt %). Thus, on
a gravimetric basis, mmen-CuBTTri adsorbs nearly 3.5 times as much
CO.sub.2 at the relevant pressures. Volumetrically, however,
mmen-CuBTTri adsorbs about 4.7 times more CO.sub.2 at 0.15 bar than
CuBTTri. The difference between gravimetric and volumetric
densities is a direct consequence of the increased mass of the
appended framework over the unappended material.
[0082] At 25.degree. C., mmen-CuBTTri adsorbs less N.sub.2 than
CuBTTri at all pressures between 0.0 and 1.1 bar. This is due to
the reduction in specific surface area upon incorporation of mmen,
with the BET surface area of 870 m.sup.2/g for mmen-CuBTTri being
roughly half of the 1770 m.sup.2/g observed for CuBTTri. The
additional polarizing sites in mmen-CuBTTri enhance N.sub.2
adsorption less than the decreased surface area diminishes N.sub.2
adsorption. The opposite trend was observed for CO.sub.2
adsorption. Enhanced adsorption of only one gas is a defining
characteristic of chemisorption. In contrast, frameworks replete
with open metal cation sites can be expected to polarize all gases
more effectively, including N.sub.2, accounting for the
substantially greater N.sub.2 adsorption in Mg.sub.2(dobdc)
relative to mmen-CuBTTri.
[0083] The selectivity (S) for adsorption of CO.sub.2 over N.sub.2
in mmen-CuBTTri was estimated from the single-component isotherm
data. For CO.sub.2 capture, this value typically reports the ratio
of the adsorbed amount of CO.sub.2 at 0.15 bar to the adsorbed
amount of N.sub.2 at 0.75 bar; the value is normalized for the
pressures chosen. The values are derived from an approximate flue
gas composition of 15% CO.sub.2, 75% N.sub.2, and 10% other gases,
at a total pressure of 1 bar. Pure-component isotherm
selectivities, which frequently are calculated from the excess
adsorption data directly measured by gas adsorption, can be
misleading. The adsorption selectivity, S.sub.IAST, was therefore
modeled by applying the Ideal Adsorbed Solution Theory (IAST) to
the calculated absolute adsorption isotherms. The accuracy of the
IAST procedure has been established for the adsorption of a wide
variety of gas mixtures in different zeolites, as well as CO.sub.2
capture in metal-organic frameworks. For mmen-CuBTTri, SIAST values
were calculated to be 327, 200, and 123 at 25.degree. C.,
35.degree. C., and 45.degree. C., respectively. The selectivity
that was observed at 25.degree. C. is one of the highest values
reported for a metal-organic framework.
Example 3
[0084] To further characterize the mmen-CuBTTri frameworks, the
isosteric heat of adsorption and working capacity of the materials
were analyzed. Utilizing a dual-site Langmuir adsorption model,
isosteric heats of adsorption were calculated for CO.sub.2 in
mmen-CuBTTri and compared to those obtained from data for bare
CuBTTri, which were fit using a single-site Langmuir model to give
a value of -24 kJ/mol. The N.sub.2 adsorption isotherm for
mmen-CuBTTri was also fit to a single-site Langmuir model,
resulting in a calculated isosteric heat of adsorption of -15
kJ/mol.
[0085] The isosteric heat of CO.sub.2 adsorption in mmen-CuBTTri
approaches -96 kJ/mol at zero coverage, corresponding to the
largest value yet reported for CO.sub.2 adsorption in a
metal-organic framework. For comparison to mmen-CuBTTri, the heat
of adsorption for en-CuBTTri was recalculated with the same
dual-site Langmuir model. Because this model incorporates absolute
adsorption, direct comparisons between the two different models are
not possible. From the dual-site Langmuir model, the isosteric heat
of CO.sub.2 adsorption in en-CuBTTri was calculated to be -78
kJ/mol at zero coverage, nearly 20 kJ/mol lower in magnitude than
the heat calculated for mmen-CuBTTri. Preferably, the isosteric
heat of CO.sub.2 adsorption of the material is greater than -70
kJ/mol at zero coverage as determined by the Clausius-Clapeyron
relation.
[0086] Accordingly, mmen-CuBTTri has a significantly larger number
of free amines available to bind guest CO.sub.2 molecules. Because
isosteric heats correspond to the average of all adsorption sites
potentially populated at a specific coverage level, at zero
coverage there is a higher probability of the CO.sub.2 molecule
adsorbing onto an amine in mmen-CuBTTri compared with
en-CuBTTri.
[0087] Despite the large binding enthalpy between alkylamines and
CO.sub.2, the substantial decrease in the -Q.sub.st data with
loading indicates that many weaker adsorption sites are also being
sampled by the CO.sub.2 molecules under the conditions probed.
While amine sterics may play a role in the improved adsorption
properties of mmen-CuBTTri, it is not possible to make comparisons
between isosteric heats of adsorption as a function of amine
sterics because of the differences in amine loading levels.
[0088] The significantly greater working capacities of strongly
binding adsorbents may lead to materials that are less expensive to
operate than those that have smaller working capacities. Solid
adsorbents with large isosteric heats of adsorption have
considerable advantages including high selectivity and high
capacity for CO.sub.2 at low partial pressures; however, they are
often believed to be the most difficult to regenerate.
Regeneration, however, is very dependent on the method best suited
to a given material. For carbon capture from flue gas streams,
vacuum and temperature swing adsorption methodologies are the ones
most frequently envisioned. Vacuum swing adsorption can best be
approximated by the difference in capacities between the adsorption
and desorption pressures. For mmen-CuBTTri, a 7 wt % working
capacity between 0.15 and 0.02 bar at 25.degree. C. was calculated.
For temperature swing methods, adsorbents with high heats of
adsorption may prove to be better candidates than materials with
moderate heats of adsorption. This is because the capacities of
adsorbents with high heats of adsorption are more dependent on
temperature than materials with smaller heats of adsorption.
[0089] The cyclability of the mmen-CuBTTri material as a CO.sub.2
adsorbent was evaluated using a combined temperature swing and
nitrogen purge approach. Utilizing a thermogravimetric analyzer, a
mixture of 15% CO.sub.2 in N.sub.2 was introduced into the furnace
for 5 minutes at 25.degree. C. It was observed that the sample mass
increased by nearly 7% upon introduction of the mixed gas, due to
strong adsorption of CO.sub.2, even in the dilute mixture. The
adsorbent was then regenerated by changing the flow to a pure
N.sub.2 stream followed by rapid ramping of the furnace at
5.degree. C./min to 60.degree. C. The sample was held for 2 minutes
at 60.degree. C., and then cooled at 5.degree. C./min to 25.degree.
C. The temperature was allowed to stabilize for 2 minutes, followed
by the reintroduction of the 15% CO.sub.2 in N.sub.2 mixture. The
27 minute cycling procedure was repeated 72 times, with no apparent
change in capacity as shown in FIG. 7. The kinetics of the
adsorption are sufficiently quick that little additional CO.sub.2
is adsorbed after the first few minutes, and even shorter cycle
times could be utilized with only small reductions in capacity.
Similarly, complete desorption is realized prior to the end of each
2 minute isotherm. Importantly, the cycling capacity of
mmen-CuBTTri under dry conditions is comparable to or even greater
than the working capacity of a 30% MEA solution, which is
frequently reported as 5.5 wt %.
Example 4
[0090] Heats of adsorption approaching -100 kJ/mol and highly
specific interactions are indicative of chemisorptive processes. In
the absence of water, it is believed that the electrophilic
CO.sub.2 molecule is accepting electron density from the lone pair
of the free amine of mmen, forming a zwitterionic carbamate.
Previous spectroscopic studies of CO.sub.2 binding in amine
containing metal-organic frameworks have investigated only less
basic aromatic amines. For example, it was recently shown in
NH.sub.2-MIL-53(AI) that the amine was not directly interacting
with the CO.sub.2, but rather, other physisorptive processes
account for the favorable adsorption characteristics of the
material.
[0091] Diffuse Reflectance Infrared Fourier Transform Spectroscopy
(DRIFTS) measurements were performed on mmen-CuBTTri using a
high-pressure (0-3 bar) gas cell to confirm and characterize the
proposed chemisorptive process. Infrared spectra were collected on
a Perkin Elmer Spectrum 400 FTIR spectrometer equipped with an
attenuated total reflectance accessory (ATR). For Diffuse
Reflectance Infrared Fourier Transform Spectroscopy (DRIFTS)
spectra, the FTIR spectrometer was equipped a Barrick Praying
Mantis Diffuse Reflectance accessory and a high-pressure gas cell
with temperature control. 5% CO.sub.2 in Be (Praxair certified
standard BE CD5C-K) and a vacuum were attached to the high-pressure
cell. Gas was slowly introduced into an evacuated cell containing
mmen-CuBTTri prepared in a N.sub.2 filled glovebag. Maximum
pressure delivered to the cell was 1.5 bar above atmospheric
pressure. Following adsorption, the sample was regenerated at
60.degree. C. under dynamic vacuum for two hours.
[0092] FIG. 3 plots the infrared absorbance of mmen-CuBTTri under
various pressures of CO.sub.2 in a 5% CO.sub.2/95% He gas mixture
in the high-pressure DRIFTS cell. Under dry conditions, the N--H
stretch of mmen is apparent at 3283 cm.sup.-1 (vertical dashed
line) on a fully evacuated sample is shown at plot 28. The reported
frequency for the N--H stretch in free mmen is 3279 cm.sup.-1.
Dilute CO.sub.2 in He was slowly introduced into the cell at plot
30 up to a dynamic pressure of 1.5 bar at plot 32. Upon saturation,
a total disappearance of the N--H band at 3283 cm.sup.-1 is clearly
observed with the introduction of 5% CO.sub.2 in He in the cell at
increasing pressures. Upon regeneration of the solid under vacuum
and heating at 60.degree. C. at plot 34, the N--H stretching band
returned. A sharp band at 1386 cm.sup.-1 also appears in the
spectra as CO.sub.2 is introduced; diffuse bands near 1669, 1487,
and 1057 cm.sup.-1 are also apparent in addition to changes in the
fingerprint region.
[0093] Water was strictly excluded from the material for the DRIFTS
measurements to eliminate the possibility of carbonate formation.
Because the amines are tethered to the framework and well
separated, we do not believe that it is possible for two amines to
be concertedly interacting with a single CO.sub.2 molecule. Similar
measurements have been performed on other porous materials, most
notably amine-grafted mesoporous silicas. In these materials,
however, two amines can often act concertedly and the experiments
frequently incorporated water vapor, making direct comparisons
difficult. It has been previously reported, however, in dry amino
acid-based ionic liquids that zwitterionic CO.sub.2-species exhibit
sharp infrared band near 1660 cm.sup.-1. From the large calculated
heat of adsorption and observed band changes in the infrared region
upon CO.sub.2 addition, it is believed that the primary mechanism
of CO.sub.2 adsorption at low pressures is the chemisorption of
CO.sub.2 gas onto mmen molecules resulting in the formation of
zwitterioinc carbamates or carbamic acid.
Example 5
[0094] The steric effect upon diamine incorporation was also
evaluated. The framework CuBTTri was additionally modified with N
methylethylenediamine (men), an asymmetric diamine with one primary
and one secondary amine. The adsorption isotherms of mmen-CuBTTri
for CO.sub.2 at 298 K (squares), 308 K (circles) and 318 K
(triangles) is shown in FIG. 4 as a baseline for comparison. FIG. 5
shows N.sub.2 adsorption isotherms at 298 K (squares), 308 K
(circles) and 318 K (triangles) temperatures for mmen-CuBTTri.
[0095] Relative to the performance of mmen-CuBTTri, men-CuBTTri
performs more similarly to en-CuBTTri, for which only a small
enhancement in CO.sub.2 uptake was observed at very low pressures.
A small improvement in performance was, however, realized through
the use of men over en.
[0096] The significantly greater adsorption of CO.sub.2 in
mmen-CuBTTri is attributable to the larger number of amines that
are accessible to guest CO.sub.2 molecules. There are two primary
factors affecting the incorporation of primary amines into CuBTTri.
First, the best fits to the elemental analysis data indicate at
least two times as many diamine molecules were incorporated into
mmen-CuBTTri versus the en- and men-analogues. A reasonable
explanation for the higher incorporation of mmen into the framework
is the formation of a weaker coordinate bond between the secondary
amine on mmen and a framework Cu.sup.2+ ion compared with the
relatively stronger coordinate bond formed between a primary amine
and a Cu.sup.2+ ion. The significantly greater reversibility of
mmen binding with Cu.sup.2+ imparts it with a faster diffusion rate
through the pores such that mmen molecules that bonded with copper
sites near pore openings were labile in the hot hexane solution
employed for grafting. These diamines were capable of migrating
deeper into pores to achieve high surface coverage. In the case of
primary amines, the irreversibility of the coordinate bond at the
synthesis conditions surveyed severely limited the diffusion of en
and men into the pores. Once appended, additional diamines could
only diffuse through constricted pores to reach interior metal
sites. Note that in men-CuBTTri, the primary amine end of men is
coordinated to the metal centers while the sterically hindered
secondary amine is available to interact with guest molecules in
the pores. Increased reversibility of en and men binding was sought
through the use of higher boiling solvents and lower
concentrations; however, grafting temperatures that significantly
exceeded 100.degree. C. resulted in the decomposition of the
framework.
[0097] Second, based upon the calculated heats of adsorption for
en- and men-CuBTTri, the number of amines that were strongly
adsorbing CO.sub.2 was significantly less than the number of amines
that were appended in each framework. This is because not all of
the amines in the en- and men-frameworks bonded to open metal
sites. Grafted amines severely reduced the pore diameters, slowing
diffusion rates as previously discussed. Some of these pores then
became blocked by the excess amines. Amines and other adsorption
sites beyond these blockages were inaccessible to guest gases. This
was confirmed by the low surface areas calculated for en- and
men-CuBTTri. By comparison, the surface area for mmen-CuBTTri was
significantly higher despite the greater number of total amines
calculated to be within the framework.
Example 6
[0098] Isosteric heats of adsorption are commonly calculated after
fitting adsorption equilibrium data to the Virial equation. FIG. 6
is a graph of isosteric heats of adsorption for mmen-CuBTTri
calculated from the virial method (circles) and the dual-site
Langmuir method (squares). FIG. 6 overlays the isosteric heats of
adsorption calculated for mmen-CuBTTri using both the dual-site
Langmuir and the Virial methods. At zero coverage, the Virial
method gives a significantly lower magnitude for the heat of
adsorption: -66 kJ/mol.
[0099] However, inflections in the isotherms tor mmen-CuBTTri are
not accurately modeled with the Virial method. It is therefore
believed that the values of the dual-site model are significantly
more accurate at low CO.sub.2 loadings. In contrast, at
intermediate loadings there is good agreement between the dual-site
Langmuir and Virial fits. While mmen-CuBTTri exhibits a large heat
of adsorption at zero loading, heats of adsorption at intermediate
loadings are also important for CO.sub.2 capture. At a loading of
2.4 mmol/g, the approximate capacity of mmen-CuBTTri for CO.sub.2
at 0.15 bar, the isosteric heat of adsorption was calculated to be
about -45 kJ/mol by both the dual-site Langmuir and Virial models.
Hence, in CO.sub.2 capture applications, the average enthalpy of
adsorption for CO.sub.2 would be significantly less than the -96
kJ/mol value calculated for very low coverage levels. This has
important implications for adsorbent regeneration.
[0100] The measured experimental data on pure component isotherms
for CO.sub.2 and N.sub.2, in terms of excess loadings, were first
converted to absolute loading using the Peng-Robinson equation of
state for estimation of the fluid densities. The pore volume of
mmen used for this purpose was 0.363 cm.sup.3/g, based on the
N.sub.2 adsorption data at 77 K. The pore volume obtained was 51%
that of bare CuBTTri.
[0101] The absolute component loadings were fitted with either a
single-site Langmuir model or a dual-site Langmuir model. For
N.sub.2/mmen there are no discernible isotherm inflections and
therefore the single-site Langmuir model was used for isotherm
fitting. The single-site Langmuir model is also adequate for
fitting the isotherm data for CO.sub.2 in "bare" CuBTTri. In order
to fit the experimental data for adsorption of CO.sub.2 in
mmen-CuBTTri, the dual-site Langmuir model was employed.
[0102] Due to their high surface areas and low bulk densities,
these materials demonstrate remarkable working capacities for
sequestering carbon dioxide, making them ideal for use in large
scale processing plants and a great improvement over current
adsorbents. The successful implementation of these new adsorbents
could both reduce the substantial energy cost of hydrogen
purification and reduce or eliminate CO.sub.2 emissions in the
generation of electricity from coal or syngas.
Example 7
[0103] To further demonstrate the broad functionality of the
metal-organic framework family M.sub.2(dobpdc) (M=Mg, Mn, Fe, Co,
Cu, and Zn), the framework was used for the separation of CO.sub.2
from mixed nitrogen gases. The M.sub.2(dobpdc) structure type
features approximately 18 .ANG.-wide channels and exhibits
exceptional CO.sub.2 adsorption properties upon functionalization
with mmen. Several different metal organic frameworks were prepared
for comparison testing.
[0104] Referring to FIG. 8A and FIG. 8B, one synthesis scheme for
the mmen-M.sub.2-(dobpdc) functionalized framework is illustrated.
As shown schematically in FIG. 8A, the initial framework is
produced from H.sub.4dobpdc
(4,4'-Dihydroxy-(1,1'-biphenyl)-3,3'-dicarboxylic Acid).
H.sub.4dobpdc was produced by adding 4,4''-dihydroxybiphenyl (1.16
g, 6.24 mmol), KHCO.sub.3 (2.00 g, 20.0 mmol), dry ice (4 g), and
1,2,4-trichlorobenzene (3 mL) to a PTFE insert within a steel acid
digestion bomb (23 mL) and heated at 255.degree. C. for 17 hours.
After cooling to room temperature, the mixture was collected via
vacuum filtration and washed with diethyl ether. The solid was
suspended in 300 mL of distilled water, and filtered again. To the
filtrate, neat HCl was slowly added until a pH between 1 and 2 was
reached. The resulting crude product was collected via filtration.
Recrystallization using 50 mL of acetone and 50 mL of water per
gram of crude material afforded 0.68 g (40%) of pure product as a
white powder.
[0105] Reaction of H.sub.4dobpdc with ZnBr.sub.2.2H.sub.2O or
MgBr.sub.2.6H.sub.2O in 1:1 DEF: EtOH produces
Zn.sub.2(dobpdc)(DEF).sub.2.DEF.H.sub.2O (DEF-1) and
Mg.sub.2(dobpdc)(DEF).sub.2.DEF.sub.1.5.H.sub.2O (DEF-2),
respectively as seen in FIG. 8A. DEF-1 was produced by mixing
H.sub.4dobpdc (4.0 mg, 0.015 mmol), ZnBr2.2H.sub.2O (8.9 mg, 0.034
mmol), and 0.5 mL of mixed solvent (1:1 DEF:EtOH;
DEF=N,N'-diethylformamide) a 2-mL Pyrex tube. The tube was sealed
and placed in a preheated oven at 100.degree. C. After 72 hours,
needle-shaped, colorless crystals had formed. The crystals were
isolated by filtration and washed with hot DEF to afford 3.7 mg
(35%) of product.
[0106] Similarly, DEF-2 was produced by loading H.sub.4dobpdc (24
mg, 0.088 mmol), MgBr.sub.2.6H.sub.2O (60 mg, 0.21 mmol), and 3 mL
of solvent (1:1 DEF:EtOH) into a 10 ml Pyrex cell and sealed with a
PTFE cap. The mixture was irradiated in a microwave reactor (CEM
Discover) for 30 minutes at 120.degree. C. After 30 minutes, the
solution was cooled and the resulting solid was collected via
filtration and washed with hot DEF. The solid was dried under
vacuum to yield 57.5 mg (95%) of product as a white powder.
[0107] Upon exposure of DEF-2 to atmospheric air, the white powder
turns blue. Amine functionalization, however, appears to enhance
framework stability, because no similar degradation was observed
for mmen-2 upon exposure to air for one week.
[0108] In the crystal structure of DEF-1, four different
dobpdc.sup.4- ligands and one DEF molecule are bonded to each
Zn.sup.2+ ion in a distorted octahedral geometry. There are three
unique 0 donor types from the dobpdc.sup.4- ligand: bridging
(.mu..sub.2) aryloxide 0 atoms (01), bridging (.mu..sub.2)
carboxylate O atoms (O.sub.2), and non-bridging carboxylate O atoms
(O.sub.3). The equatorial plane of each Zn.sup.2+ is composed of
two trans-disposed O1 ligands from different linkers, one O3 donor
atom, and one O2 donor atom. An O2 donor atom occupies one axial
coordination site, while the other axial site is occupied by an O
donor atom from DEF, the reaction solvent. This coordination mode
results in the formation of helical chains of Zn.sup.2+ atoms
running along the c axis of the crystal. The resulting framework
consists of a honeycomb lattice of hexagonal, one-dimensional
channels approximately 18 A in width. Bound DEF molecules occupy
the Zn.sup.2+ coordination sites along the corners of hexagonal
channel walls. Powder X-ray diffraction (PXRD) data indicate DEF-2
to be isostructural with DEF-1.
[0109] Heating DEF-1 or DEF-2 at 420.degree. C. for 65 minutes in
vacuo yielded the fully activated adsorbent Mg.sub.2(dobpdc) or
Zn.sub.2(dobpdc) respectively. Heating DEF-2 at 420.degree. C. for
65 min under dynamic vacuum, removed the DEF molecules bound to the
metal atoms, completely activating the material and generating open
Mg.sup.2+ coordination sites. Such extreme thermal treatment was
necessary because soaking in methanol at 100.degree. C. for 20
hours did not lead to exchange of the bound DEF molecules. The
porosity of activated DEF-2 was confirmed via N.sub.2 adsorption at
77 K, resulting in a BET surface area of 3270 m.sup.2/g. Note that,
in line with the expanded structure, this is significantly greater
than the BET surface area of 1495 m.sup.2/g reported for
Mg.sub.2(dobdc).
[0110] The synthesis and structure of mmen-Mg.sub.2(dobpdc) or
mmen-2 is depicted schematically at the right of FIG. 8A and FIG.
8B. An activated sample of DEF-1 of DEF-2 was suspended in hexanes
and an excess of mmen was added. Specifically, a 77 mg, 0.24 mmol
sample of activated DEF-2 was immersed in anhydrous hexane, and 20
equivalents of N,N'-dimethylethylenediamine (mmen, 0.53 mL, 4.8
mmol) was added. The suspension was stirred for one day, filtered,
and rinsed copiously with hexanes. The solid was then evacuated of
residual solvents at 100.degree. C. for 24 h to afford 87 mg (77%)
of product as a gray-white powder.
[0111] As shown by powder X-ray diffraction, framework
crystallinity was not significantly affected by activation or
subsequent amine functionalization. A much reduced BET surface area
of 70 m.sup.2/g was calculated for mmen-2, while DFT pore size
distributions indicated a reduction in average pore size.
Example 8
[0112] CO.sub.2 adsorption isotherms were taken of the mmen-2
functionalized frameworks and the bare Mg.sub.2(dobpdc) framework
for comparison. Isosteric heats of adsorption for Mg.sub.2(dobpdc)
were calculated to be -44 kJ/mol at low coverage. This value is 42
kJ/mol higher in magnitude than the -42 kJ/mol previously reported
for the analogous Mg.sub.2(dobdc) framework.
[0113] The adsorption capacity of Mg.sub.2(dobpdc) at 25.degree. C.
is 4.85 mmol/g (13.8 wt %) and 6.42 mmol/g (20.0 wt %) at 0.15 and
1 bar, respectively. The capacity of Mg.sub.2(dobpdc) for CO.sub.2
at 0.15 bar exceeds the capacity of most metal-organic
frameworks.
[0114] The alkylamine-functionalized metal-organic framework mmen-2
displayed an extremely high affinity for CO.sub.2 at
extraordinarily low pressures. The CO.sub.2 adsorption isotherms
obtained at 25, 50, and 75.degree. C. are presented in FIG. 9. At
25.degree. C. and 0.39 mbar, near the current partial pressure of
CO.sub.2 in Earth's atmosphere, the compound adsorbed 2.0 mmol/g
(8.1 wt %), which is 15 times the capacity of Mg.sub.2(dobpdc). At
the much higher pressure of 5 mbar, the median partial pressure of
CO.sub.2 within the International Space Station, the framework
adsorbed 2.6 mmol/g (10.3 wt %). For comparison, zeolite 5 A, which
is currently used aboard the station to adsorb CO.sub.2, adsorbs
0.85 mmol/g (3.6 wt %, crystallographic volumetric capacity 1.3
mmol/cm.sup.3) at 5 mbar.
[0115] At 25.degree. C., the CO.sub.2 adsorption in mmen-2 reaches
3.14 mmol/g (12.1 wt %) at 0.15 bar and 3.86 mmol/g (14.5 wt %) at
1 bar. Remarkably, its CO.sub.2 uptake at 1 bar and 25.degree. C.
exceeds the amount of N.sub.2 adsorbed at 77 K. Thus, the low
surface area measured at 77 K does not appear to accurately reflect
the surface area accessible to CO.sub.2. On a per mass basis, the
amine-functionalized framework adsorbed less CO.sub.2 than
Mg.sub.2(dobpdc) at pressures higher than ca. 0.1 bar. While the
decreased surface area of mmen-2 may limit its capacity at
super-atmospheric pressures, the large density difference between
the two frameworks is primarily responsible for the lower
gravimetric capacity of mmen-2. Crystallographic densities of 0.58
and 0.86 g/cm.sup.3 were calculated for Mg.sub.2(dobpdc) and
mmen-2, respectively. For adsorbents of the same structure and
widely different densities, volumetric capacities better represent
CO.sub.2 adsorption performance. At 0.15 bar, Mg.sub.2(dobpdc) and
mmen-2 adsorb 2.1 and 2.7 mmol/cm.sup.3, respectively, while at 1
bar, the capacities of both adsorbents are 3.3 mmol/cm.sup.3.
[0116] For stationary applications like CCS, the greater volumetric
capacity of mmen-2 makes it the superior adsorbent. Based upon the
calculated number of dangling amine groups in mmen-2, a loading of
3.4 mmol/g would correspond to one CO.sub.2 per amine, yet uptake
of only ca. 2.2 mmol/g was observed. Here, pore blockages, hydrogen
bonded amines, or cooperative binding mechanisms between two amines
and one CO.sub.2 may be limiting the accessible stoichiometry of
mmen-2. Thus, significant additional capacity improvements might be
realized in the material if conditions can be identified for
appending one mmen per magnesium and binding one CO.sub.2 molecule
per dangling amine.
[0117] Isosteric heat of adsorption calculations were hindered by
the presence of a prominent step in the isotherms at low pressures
and convex to the pressure axis. Generally, continuous mathematical
functions are used to model experimental isotherms, which then
become the input parameters for the Clausius-Clapeyron relation.
Since mathematical modeling of the CO.sub.2 isotherms of mmen-2
with continuous equations over the entire pressure range were
unavailable, each isotherm was modeled with two Langmuir-Freundlich
equations. Data sets corresponding to the adsorption regions before
and after the steps were compiled and then modeled individually.
Isosteric heats of adsorption for mmen-2 were calculated from the
25, 50, and 75.degree. C. isotherm models. At low loadings, heats
significantly lower than those expected for chemical adsorption of
CO.sub.2 onto an amine were calculated. However, calculated heats
quickly approached and maintained a value of -71 kJ/mol, which
likely corresponds to the chemical adsorption of CO.sub.2 onto the
free amine of mmen. Here, a carbamate with a weak C--N bond is
probably formed through interaction the lone pair of the free amine
of mmen and the electrophilic carbon of CO.sub.2.
[0118] In situ diffuse reflectance infrared Fourier transform
spectroscopy (DRIFTS) was employed to probe the chemical nature of
adsorbed CO.sub.2. At 25.degree. C., 1 bar of 5% CO.sub.2 in Helium
was introduced into an air-tight gas cell containing a sample of
activated mmen-2. The difference spectrum between mmen-2 under a 1
bar atmosphere and the activated framework under vacuum was
obtained. A prominent loss peak was observed at 3316 cm.sup.-1 and
assigned to the NH stretch of free mmen-2, and is indicative of
chemical adsorption of CO.sub.2 onto amines. Gain bands observed at
1702 cm.sup.-1 and 1531 cm.sup.-1 likely correspond to carbamate
C.dbd.O and CHN group stretches, respectively.
[0119] Recent work on alkylamine grafted silica surfaces have
suggested that chemical adsorption of CO.sub.2 onto alkylamines is
not possible without neighboring amines or surface hydroxyl groups
to stabilize the resulting carbamates; ammonium carbamates or
surface bonded carbamates are formed, respectively. The formation
of surface bonded carbamates in mmen-2 is unlikely due to a lack of
surface hydroxyl groups, and the broad NH stretches expected for
ammonium cations are not definitively resolvable from the DRIFTS
difference spectrum. Furthermore, the slow reversibility of mmen-2
at room temperature appears to preclude the formation of ammonium
carbamates, which have been reported to desorb CO.sub.2 from
primary amines readily at room temperature.
[0120] The step observed in each isotherm marks the pressure at
which CO.sub.2 adsorption becomes dominated by chemisorption.
Interestingly, the step moves to significantly higher pressures as
the adsorption temperature increases. The location of the step is
modeled well by a simple Clausius-Clapeyron relation, which
predicts how isotherms move as a function of temperature. The
existence of the step, however, is unexpected in a strongly
adsorbing material with large pores, and can best be explained by
the surprising conclusion that weaker adsorption sites are
energetically favored over amine adsorption sites at low coverage.
This suggests that adsorption of CO.sub.2 onto mmen is disfavored
at low adsorptive concentrations (the density of gas phase CO.sub.2
in the pores) because of the large positive entropy associated with
reorganization of the amines, as required to form a chemical bond
with CO.sub.2.
Example 9
[0121] To evaluate the performance of mmen-2 as a regenerable
adsorbent for CO.sub.2 capture from air, thermogravimetric analysis
(TGA) was utilized to monitor sample mass under dynamic
environments. The changes in sample mass (normalized to the mass of
the framework under N.sub.2 at 25.degree. C.) while simulated air
containing 390 ppm CO.sub.2 was flowed over the sample were
obtained. Despite the very low concentration of CO.sub.2, a 4.6% mc
(% mc=percent mass change; 1.05 mmol/g; 4.4 wt %) was realized
after 60 min.
[0122] Thermogravimetric analyses were carried out at ramp rates
between 5 and 10.degree. C./min under a nitrogen flow with a TA
Instruments TGA Q5000 (Ver. 3.1 Build 246) or a Scinco TGA N-1000.
Carbon dioxide cycling experiments were performed using a 15%
CO.sub.2 in N.sub.2 (Praxair NI-CD15C-K), 390 ppm CO.sub.2 in air
(Praxair AI-CD-390C-K; 390 ppm CO.sub.2, 21% O.sub.2, balance
N.sub.2), CO.sub.2 (Praxair 99.998%) and N.sub.2 (Praxair, 99.9%).
A flow rate of 25 mL/minute was employed for all gases. Prior to
cycling, the sample was activated by heating at 150.degree. C. for
1 hour. The sample mass was normalized to be 0% at the adsorption
temperature (25.degree. C. for 390 ppm CO.sub.2 and 40.degree. C.
for 15% CO.sub.2) under flowing N.sub.2 and sample mass was
normalized to be 0% at 150.degree. C. under flowing 100%
CO.sub.2.
[0123] The adsorbent was then regenerated under flowing N.sub.2 at
150.degree. C. for 30 minutes and the cycle repeated ten times with
no apparent loss of capacity. The equilibrium capacity (2.0 mmol/g,
1.72 mmol/cm.sup.3) of mmen-2 is similar to the capacities of the
very best amine-grafted silica and alumina adsorbents reported to
date. However, the kinetics of adsorption appear to be
significantly faster in mmen-2 than for amines deposited via
evaporation or polymerization methodologies. For example, while the
pseudo-equilibrium capacity of an outstanding poly(ethyleneimine)
impregnated silica gel was reported to be 2.4 mmol/g, it took
nearly 200 minutes for the silica based adsorbent to realize 4.6%
mc, the capacity of mmen-2 for CO.sub.2 after only 60 minutes. The
easily accessed amines within mmen-2 appear to enhance adsorption
rates greatly, enabling rapid adsorption-desorption cycles to be
utilized.
Example 10
[0124] The capabilities of mmen-2 as an adsorbent for removing
CO.sub.2 from the flue gas of coal-fired power stations were also
investigated. The dynamic cycling behavior of mmen-2 under the
relevant, dry conditions: 15% CO.sub.2 in N.sub.2 flowing over the
solid at 40.degree. C. was also investigated.
[0125] After adsorbing CO.sub.2 for 15 minutes, the sample was
heated at 120.degree. C. for 15 minutes under N.sub.2. A capacity
of 11.1% mc (2.52 mmol/g, 9.9 wt %) relative to the sample mass of
mmen-2 under N.sub.2 at 40.degree. C. was realized. After 50
cycles, only a 0.2% mc capacity loss was observed. Longer
adsorption and desorption times did not significantly improve the
cycling capacity of the material, nor did higher desorption
temperatures. It was noted that the apparent capacity of mmen-2
greatly exceeds the ca. 2 wt % working capacity of aqueous
monoethanolamine (MEA) scrubbers, which would likely swing between
the same adsorption and desorption temperatures.
[0126] If captured CO.sub.2 is to be sequestered, high purity
CO.sub.2 is essential. To desorb the ca. 98% pure CO.sub.2 adsorbed
onto mmen-2, a N.sub.2 or air purge cannot be utilized to strip the
adsorbent bed. Hence, to approximate the working capacity of mmen-2
using a temperature swing without a N.sub.2 purge, a pure CO.sub.2
purge was utilized instead. A 7.8% mc (1.8 mmol/g, 7.2 wt %) was
realized when 15% CO.sub.2 in N.sub.2 at 40.degree. C. was desorbed
with 100% CO.sub.2 at 150.degree. C.
[0127] From the discussion above it will be appreciated that the
invention can be embodied in various ways, including the
following:
[0128] 1. An adsorption material, comprising: a porous
metal-organic framework; and a plurality of ligands within the
pores of the metal-organic framework, each ligand having at least
one basic nitrogen group; wherein the basic nitrogen groups are
configured to selectively adsorb CO.sub.2 from a stream of mixed
gases at pressures below approximately 3 bar and CO.sub.2 partial
pressures between approximately 1 and 1000 mbar.
[0129] 2. The adsorption material of any previous embodiment,
wherein the metal-organic framework is a framework selected from
the group of frameworks consisting essentially of M-BTT where
(M=Ca, Fe, Mn, Cu, Co, Ni, Cr, Cd) and
(BTT=1,3,5-benzenetristetrazolate) and M-BTTri where (M=Cr, Mn, Fe,
Co, Ni, Cu) and (BTTri=1,3,5-benzenetristriazolate).
[0130] 3. The adsorption material of any previous embodiment,
wherein the metal-organic framework is a framework selected from
the group of frameworks consisting essentially of M-BTP where
(M=Co, Ni, Zn) (BTP=1,3,5-benzenetrispyrazolate) and
M.sub.3(BTC).sub.2 where (M=Cu, Cr) and
(BTC=1,3,5-benzenetriscarboxylate).
[0131] 4. The adsorption material of any previous embodiment,
wherein the metal-organic framework is a framework selected from
the group of frameworks consisting essentially of MIL-100 where
(M=Fe, Al, Cr, Ti, Sc, V) and
Ligand=BTC=1,3,5-benzenetriscarboxylate) and MIL-101 (M=Fe, Al, Cr,
Ti, Sc, V) and (Ligand=BDC=1,4-benzenedicarboxylate.
[0132] 5. The adsorption material of any previous embodiment,
wherein the metal-organic framework comprises M.sub.2(dobdc) (M=Mg,
Ca, Mn, Cr, Fe, Co, Ni, Cu, Zn)
(dobdc=2,5-dioxido-1,4-benzenedicarboxylate).
[0133] 6. The adsorption material of any previous embodiment,
wherein the basic nitrogen group is incorporated into the framework
on a ligand prior to framework formation.
[0134] 7. The adsorption material of any previous embodiment,
wherein the basic nitrogen group is incorporated into the framework
through substitution or modification of a functional group that was
bonded to a ligand prior to framework formation.
[0135] 8. The adsorption material of any previous embodiment,
wherein the basic nitrogen group is incorporated into the framework
by substitution of a ligand after framework formation with the
ligand with a basic nitrogen group.
[0136] 9. The adsorption material of any previous embodiment,
wherein the ligand comprises a first functional group reactive to
metal atoms in the metal-organic framework and a second functional
group reactive with carbon dioxide.
[0137] 10. The adsorption material of any previous embodiment,
wherein the first functional group of the ligand comprises a phenyl
group.
[0138] 11. The adsorption material of any previous embodiment,
wherein the first functional group of the ligand comprises a
carboxylate group, a triazolate group, a pyrazolate, a
tetrazolates, a pyridine, or a sulfate.
[0139] 12. The adsorption material of any previous embodiment,
wherein the ligand comprises a primary alkylamine, a secondary
alkylamine, a tertiary alkylamine, a primary imine, or a secondary
imine.
[0140] 13. The adsorption material of any previous embodiment,
wherein the metal-organic framework comprises open metal sites and
ligand occupied metal sites.
[0141] 14. The adsorption material of any previous embodiment,
wherein the adsorption material has an isosteric heat of CO.sub.2
adsorption of greater than -60 kJ/mol at zero coverage using a
dual-site Langmuir model.
[0142] 15. A method of separating a mixture stream comprising
CO.sub.2 and N.sub.2, the method comprising: contacting the mixture
stream comprising CO.sub.2 and N.sub.2 with a material comprising a
metal-organic framework, and a ligand with a basic nitrogen group;
wherein the material has an isosteric heat of CO.sub.2 adsorption
of greater than -60 kJ/mol at zero coverage using a dual-site
Langmuir model; obtaining a stream richer in CO.sub.2 as compared
to the mixture stream; and obtaining a stream richer in N.sub.2 as
compared to the mixture stream.
[0143] 16. The method as recited in any previous embodiment,
wherein the metal-organic framework is a framework selected from
the group of frameworks consisting essentially of M-BTT where
(M=Ca, Fe, Mn, Cu, Co, Ni, Cr, Cd) and
(BTT=1,3,5-benzenetristetrazolate); M-BTTri where (M=Cr, Mn, Fe,
Co, Ni, Cu) and (BTTri=1,3,5-benzenetristriazolate); M-BTP where
(M=Co, Ni, Zn) (BTP=1,3,5-benzenetrispyrazolate);
M.sub.3(BTC).sub.2 where (M=Cu, Cr) and
(BTC=1,3,5-benzenetriscarboxylate); MIL-100 where (M=Fe, Al, Cr,
Ti, Sc, V) and Ligand=BTC=1,3,5-benzenetriscarboxylate); MIL-101
where (M=Fe, Al, Cr, Ti, Sc, V) and
(Ligand=BDC=1,4-benzenedicarboxylate, and M.sub.2(dobdc) (M=Mg, Ca,
Mn, Cr, Fe, Co, Ni, Cu, Zn) where
(dobdc=2,5-dioxido-1,4-benzenedicarboxylate).
[0144] 17. The method as recited in any previous embodiment,
wherein the ligand is selected from the group of ligands consisting
essentially of a carboxylate group, a triazolate group, a
pyrazolate, a tetrazolates, a pyridine, or a sulfate.
[0145] 18. The method as recited in any previous embodiment,
wherein the ligand comprises a primary alkylamine, a secondary
alkylamine, a tertiary alkylamine, a primary imine, or a secondary
imine.
[0146] 19. A method of separating a mixture stream comprising
CO.sub.2 and other combustion gases, the method comprising:
contacting the mixture stream comprising CO.sub.2 and N.sub.2 with
a material comprising a metal-organic framework and a plurality of
ligands that have at least one basic nitrogen group; obtaining a
stream richer in CO.sub.2 as compared to the mixture stream; and
obtaining a stream richer in N.sub.2 as compared to the mixture
stream.
[0147] 20. The method as recited in any previous embodiment,
wherein the metal-organic framework and plurality of ligands
comprises mmen-CuBTTri.
[0148] 21. An adsorption material comprising: a metal-organic
framework; and a ligand with a basic nitrogen group, wherein the
adsorption material has an isosteric heat of CO.sub.2 adsorption of
greater than -80 kJ/mol at zero coverage using a dual-site Langmuir
model.
[0149] 22. The adsorption material of any previous embodiment,
wherein the basic nitrogen group is incorporated into the framework
on a ligand prior to framework formation.
[0150] 23. The adsorption material of any previous embodiment,
wherein the basic nitrogen group is incorporated into the framework
through substitution or modification of a functional group that was
bonded to a ligand prior to framework formation.
[0151] 24. The adsorption material of any previous embodiment,
wherein the basic nitrogen group is incorporated into the framework
by substitution of a ligand after framework formation with the
ligand with a basic nitrogen group.
[0152] 25. The adsorption material of any previous embodiment,
wherein the ligand comprises a carboxylate group, a triazolate
group, a pyrazolate, a tetrazolates, a pyridine, or a sulfate.
[0153] 26. The adsorption material of any previous embodiment,
wherein the ligand comprises a primary amine, a secondary amine, a
tertiary amine, a primary imine, or a secondary imine.
[0154] 27. The adsorption material of any previous embodiment,
wherein the metal-organic framework comprises open metal sites.
[0155] 28. The adsorption material of claim 1, wherein the
adsorption material has an isosteric heat of CO.sub.2 adsorption of
greater than -95 kJ/mol at zero coverage using a dual-site Langmuir
model.
[0156] 29. A method of separating a mixture stream comprising
CO.sub.2 and N.sub.2 comprising: contacting the mixture stream
comprising CO.sub.2 and N.sub.2 with a material comprising a
metal-organic framework, and a ligand with a basic nitrogen group,
wherein the material has an isosteric heat of CO.sub.2 adsorption
of greater than -80 kJ/mol at zero coverage using a dual-site
Langmuir model; obtaining a stream richer in CO.sub.2 as compared
to the mixture stream; and obtaining a stream richer in N.sub.2 as
compared to the mixture stream.
[0157] Although the description above contains many details, these
should not be construed as limiting the scope of the invention but
as merely providing illustrations of some of the presently
preferred embodiments of this invention. Therefore, it will be
appreciated that the scope of the present invention fully
encompasses other embodiments which may become obvious to those
skilled in the art, and that the scope of the present invention is
accordingly to be limited by nothing other than the appended
claims, in which reference to an element in the singular is not
intended to mean "one and only one" unless explicitly so stated,
but rather "one or more." All structural, chemical, and functional
equivalents to the elements of the above-described preferred
embodiment that are known to those of ordinary skill in the art are
expressly incorporated herein by reference and are intended to be
encompassed by the present claims. Moreover, it is not necessary
for a device or method to address each and every problem sought to
be solved by the present invention, for it to be encompassed by the
present claims. Furthermore, no element, component, or method step
in the present disclosure is intended to be dedicated to the public
regardless of whether the element, component, or method step is
explicitly recited in the claims. No claim element herein is to be
construed under the provisions of 35 U.S.C. 112, sixth paragraph,
unless the element is expressly recited using the phrase "means
for."
* * * * *