U.S. patent number 10,923,726 [Application Number 16/296,158] was granted by the patent office on 2021-02-16 for artificial solid electrolyte interphase of a metallic anode for a secondary battery including amino-functionalized carbon structures to protect the anode material, a method for producing the anode and a lithium metal secondary battery including the anode produced by the method.
This patent grant is currently assigned to Korea Institute of Science and Technology. The grantee listed for this patent is KOREA INSTITUTE OF SCIENCE AND TECHNOLOGY. Invention is credited to Won Il Cho, Van Dung Do, Min Seop Kim, Mun Sek Kim, Seung Hun Lee, In Wook Nah, In Hwan Oh.




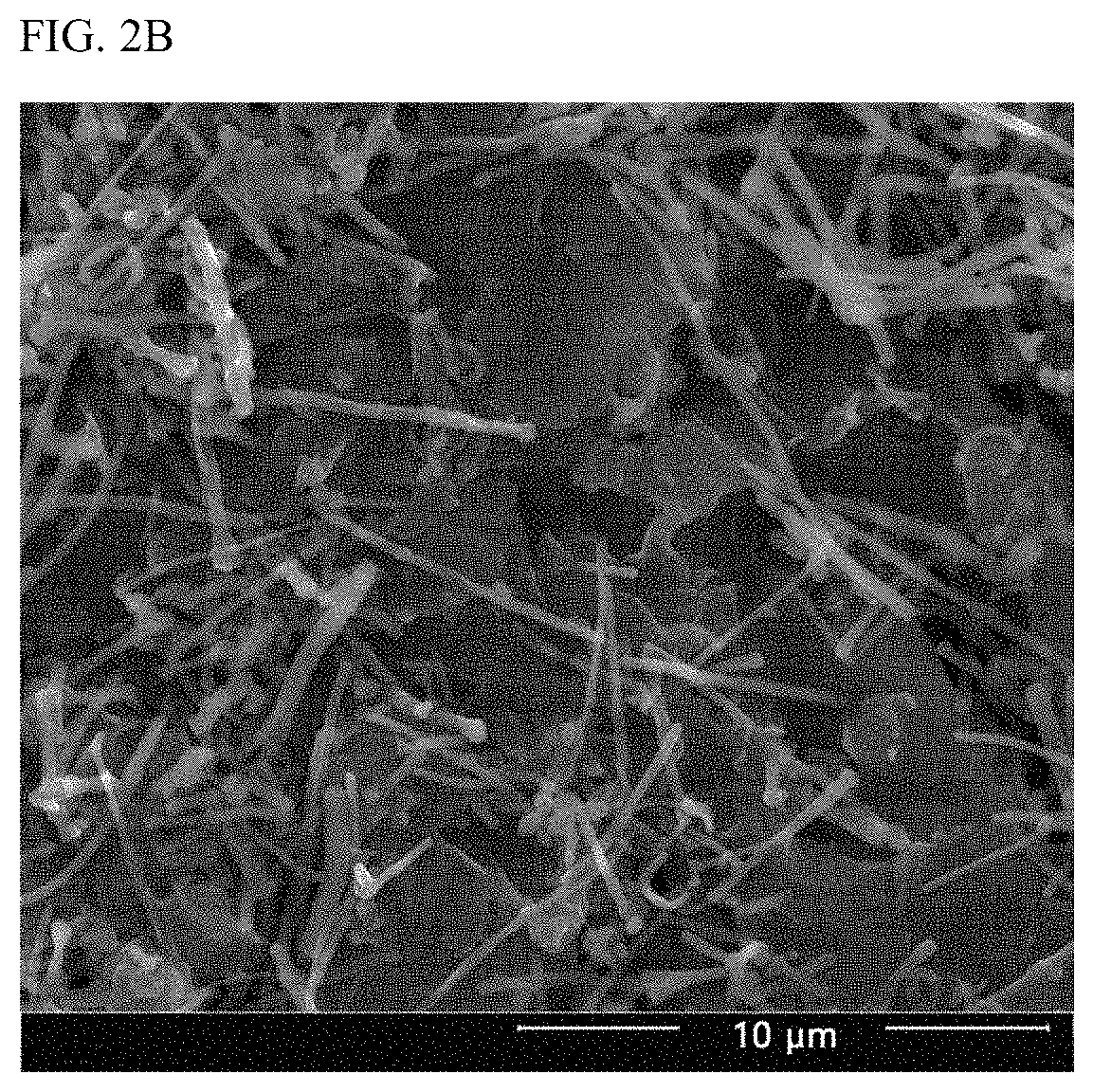

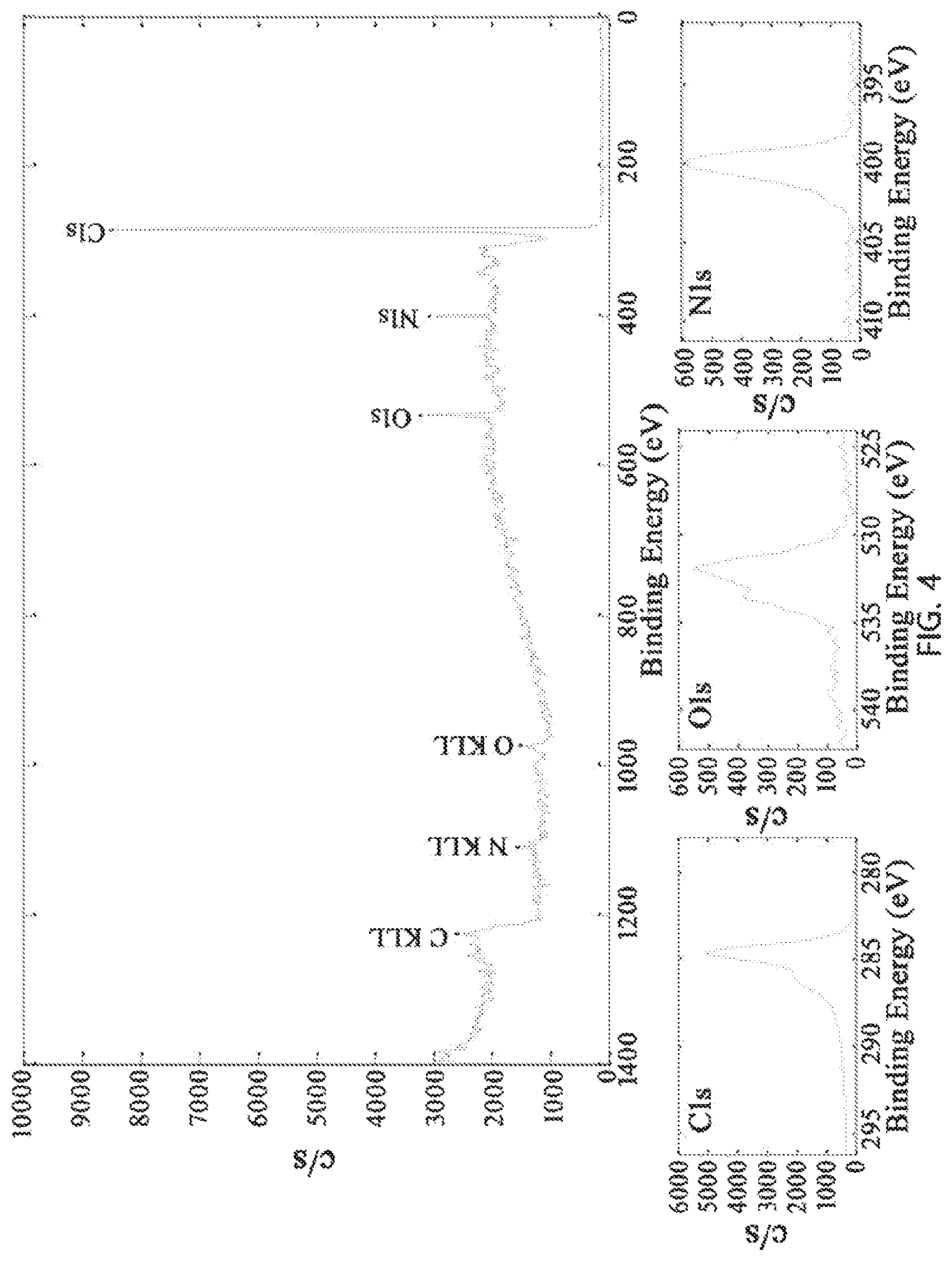



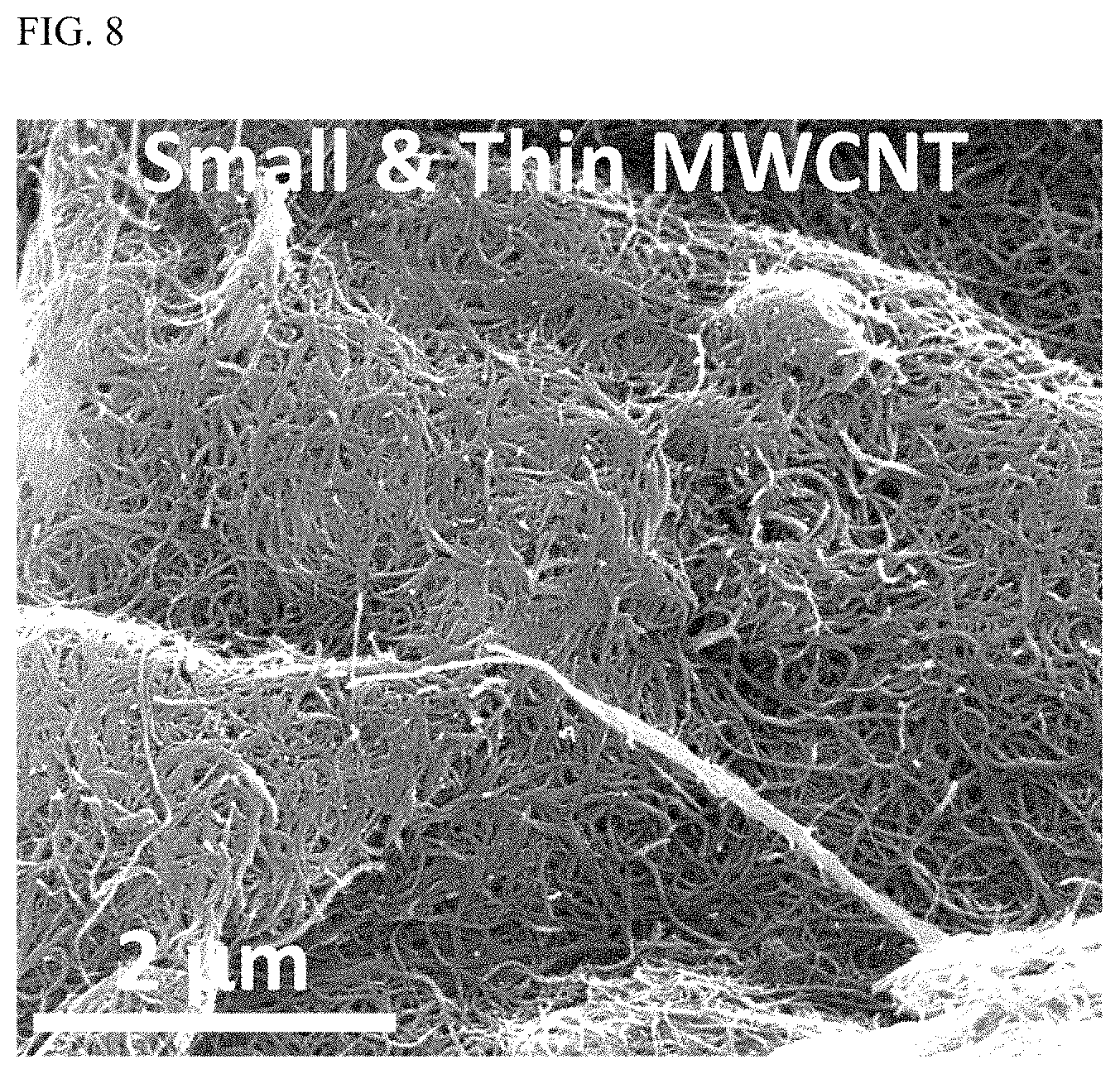
View All Diagrams
United States Patent |
10,923,726 |
Cho , et al. |
February 16, 2021 |
Artificial solid electrolyte interphase of a metallic anode for a
secondary battery including amino-functionalized carbon structures
to protect the anode material, a method for producing the anode and
a lithium metal secondary battery including the anode produced by
the method
Abstract
An artificial solid electrolyte interphase (ASEI) of an anode
for a secondary battery includes a first film composed of
amino-functionalized, reduced graphene oxide (rGO) that is
amino-functionalized by binding with polyethyleneimine present in
an amount of from 1 to 50% by weight, based on total weight of the
amino-functionalized, reduced graphene oxide (rGO) and that is
disposed in contact with an anode material to protect the anode
material; and a second film comprised of amino-functionalized,
multi-walled carbon nanotubes that is amino-functionalized by
binding with polyethyleneimine and that is stacked on the first
film. An anode of a secondary battery including the ASEI enables
rapid diffusion and stable deposition of lithium to inhibit the
formation of dendrites. In a secondary battery including the anode,
the ASEI prevents side reactions between a lithium metal anode and
the electrolyte, achieving good electrochemical stability and high
Coulombic efficiency.
Inventors: |
Cho; Won Il (Seoul,
KR), Kim; Mun Sek (Seoul, KR), Lee; Seung
Hun (Seoul, KR), Kim; Min Seop (Seoul,
KR), Do; Van Dung (Seoul, KR), Nah; In
Wook (Seoul, KR), Oh; In Hwan (Seoul,
KR) |
Applicant: |
Name |
City |
State |
Country |
Type |
KOREA INSTITUTE OF SCIENCE AND TECHNOLOGY |
Seoul |
N/A |
KR |
|
|
Assignee: |
Korea Institute of Science and
Technology (Seoul, KR)
|
Family
ID: |
1000005367694 |
Appl.
No.: |
16/296,158 |
Filed: |
March 7, 2019 |
Prior Publication Data
|
|
|
|
Document
Identifier |
Publication Date |
|
US 20190280304 A1 |
Sep 12, 2019 |
|
Foreign Application Priority Data
|
|
|
|
|
Mar 8, 2018 [KR] |
|
|
10-2018-0027172 |
Mar 14, 2018 [KR] |
|
|
10-2018-0029602 |
|
Current U.S.
Class: |
1/1 |
Current CPC
Class: |
C01B
32/174 (20170801); H01M 10/052 (20130101); H01M
4/382 (20130101); H01M 4/663 (20130101); H01M
4/131 (20130101); H01M 2004/027 (20130101); H01M
2220/10 (20130101); H01M 2220/20 (20130101); C01B
2202/06 (20130101); H01M 2004/028 (20130101); H01M
2300/0068 (20130101) |
Current International
Class: |
H01M
4/66 (20060101); H01M 4/38 (20060101); H01M
4/02 (20060101); C01B 32/174 (20170101); H01M
10/052 (20100101); H01M 4/131 (20100101) |
Field of
Search: |
;429/231.8,231.95 |
References Cited
[Referenced By]
U.S. Patent Documents
Foreign Patent Documents
|
|
|
|
|
|
|
104103791 |
|
Oct 2014 |
|
CN |
|
2007095494 |
|
Apr 2007 |
|
JP |
|
10-2013-0128273 |
|
Nov 2013 |
|
KR |
|
10-1432915 |
|
Aug 2014 |
|
KR |
|
10-1486130 |
|
Jan 2015 |
|
KR |
|
10-2016-0086194 |
|
Jul 2016 |
|
KR |
|
10-2018-0020599 |
|
Feb 2018 |
|
KR |
|
WO-2017179848 |
|
Oct 2017 |
|
WO |
|
WO-2018034526 |
|
Feb 2018 |
|
WO |
|
Other References
Ding Zhang et al., "Modified secondary lithium metal batteries with
the polyaniline-carbon nanotube composite buffer layer", Chem.
Commun., 2015, pp. 322-325, vol. 51. cited by applicant .
Lin Ma et al., "Enhanced Li-S Batteries Using Amine-Functionalized
Carbon Nanotubes in the Cathode", ACS Nano, 2016, pp. 1050-1059,
vol. 10. cited by applicant .
Dingshan Yu et al., "Self-Assembled Graphene/Carbon Nanotube Hybrid
Films for Supercapacitors", The Journal of Physical Chemistry
Letter, 2010, pp. 467-470, vol. 1. cited by applicant.
|
Primary Examiner: Cullen; Sean P
Attorney, Agent or Firm: Rabin & Berdo, P.C.
Claims
What is claimed is:
1. An artificial solid electrolyte interphase (ASEI) of an anode
for a secondary battery, comprising: a first film comprised of
amino-functionalized, reduced graphene oxide (rGO) that is
amino-functionalized by binding with polyethyleneimine present in
an amount of from 1 to 50% by weight, based on total weight of the
amino-functionalized, reduced graphene oxide (rGO) and that is
disposed in contact with an anode material to protect the anode
material; and a second film comprised of amino-functionalized,
multi-walled carbon nanotubes that is amino-functionalized by
binding with polyethyleneimine and that is stacked on the first
film.
2. The artificial solid electrolyte interphase according to claim
1, wherein each multi-walled carbon nanotube of the
amino-functionalized, multi-walled carbon nanotubes has a diameter
ranging from 50 to 300 nm and a length ranging from 3 to 50
.mu.m.
3. The artificial solid electrolyte interphase according to claim
1, wherein the second film is amino-functionalized by binding with
from 0.1 to 10% by weight of polyethyleneimine, based on total
weight of the amino-functionalized multi-walled carbon
nanotubes.
4. An anode for a secondary battery, comprising: an anode material
in the form of a foil; and an artificial solid electrolyte
interphase (ASEI) provided on the anode material to protect the
anode material and being comprised of: a first film comprised of
amino-functionalized, reduced graphene oxide (rGO) that is
amino-functionalized by binding with polyethyleneimine present in
an amount of from 1 to 50% by weight, based on total weight of the
amino-functionalized, reduced graphene oxide (rGO), and that is
disposed in contact with the anode material; and a second film
comprised of amino-functionalized, multi-walled carbon nanotubes
that is amino-functionalized by binding with polyethyleneimine and
that is stacked on the first film.
5. The anode for a secondary battery according to claim 4, wherein
each multi-walled carbon nanotube of the amino-functionalized,
multi-walled carbon nanotubes has a diameter ranging from 50 to 300
nm and a length ranging from 3 to 50 .mu.m.
6. The anode for a secondary battery according to claim 4, wherein
the second film is amino-functionalized by binding with
polyethyleneimine present in an amount of 0.1 to 10% by weight,
based on total weight of the amino-functionalized multi-walled
carbon nanotubes.
7. A secondary battery comprising the anode according to claim
4.
8. The secondary battery according to claim 7, wherein the
secondary battery is a lithium metal secondary battery wherein the
anode is comprised of lithium metal.
9. The secondary battery according to claim 7, wherein the
secondary battery has a cathode that comprises at least one
compound selected from the group consisting of lithium cobalt
oxides, lithium manganese oxides, lithium nickel cobalt aluminum
oxides, lithium nickel manganese cobalt oxides, lithium iron
phosphate oxide, and sulfur compounds, or is a porous air
electrode.
10. An electric device, comprising the anode according to claim 4,
wherein the electric device is selected from the group consisting
of electric vehicles, hybrid electric vehicles, plug-in hybrid
electric vehicles, and energy storage systems.
11. An artificial solid electrolyte interphase (ASEI) of an anode
for a secondary battery, comprising: a plurality of first films,
each first film being comprised of amino-functionalized, reduced
graphene oxide (rGO) that is amino-functionalized by binding with
polyethyleneimine present in an amount of from 1 to 50% by weight,
based on total weight of the amino-functionalized, reduced graphene
oxide (rGO), and one first film being disposed in contact with an
anode material to protect the anode material, and the plurality of
first films being stacked together as a three-dimensional network
structure; and a second film comprised of amino-functionalized,
multi-walled carbon nanotubes that is amino-functionalized by
binding with polyethyleneimine, that has a three-dimensional
structure, and that is stacked on an exposed first film of the
plurality of first films.
12. The artificial solid electrolyte interphase according to claim
11, wherein each multi-walled carbon nanotube of the
amino-functionalized, multi-walled carbon nanotubes has a diameter
ranging from 50 to 300 nm and a length ranging from 3 to 50
.mu.m.
13. The artificial solid electrolyte interphase according to claim
11, wherein the second film is amino-functionalized by binding with
polyethyleneimine present in an amount of 0.1 to 10% by weight,
based on total weight of the amino-functionalized multi-walled
carbon nanotubes.
Description
CROSS-REFERENCE TO RELATED APPLICATIONS
This application claims priorities under 35 U.S.C. .sctn. 119 to
Korean Patent Application Nos. 10-2018-0027172 and 10-2018-0029602,
filed on Mar. 8, 2018 and Mar. 14, 2018, respectively in the Korean
Intellectual Property Office, the disclosure of which is
incorporated herein by reference in its entirety.
BACKGROUND OF THE INVENTION
1. Field of the Invention
The present invention relates to an artificial solid electrolyte
interphase of an anode for a secondary battery including
amino-functionalized carbon structures to protect an anode
material, a method for producing an anode, and a lithium metal
secondary battery including an anode produced by the method. More
specifically, the present invention relates to the artificial solid
electrolyte interphase of an anode for a secondary battery
including amino-functionalized multi-walled carbon nanotubes or
amino-functionalized reduced graphene oxide to protect an
underlying anode material in the form of a foil, an anode for a
lithium metal secondary battery in which an artificial solid
electrolyte interphase is introduced to facilitate diffusion and
migration of lithium that suppress formations of Li dendrites, and
a lithium metal secondary battery using the anode in which the
artificial solid electrolyte interphase prevents side reactions
between a lithium metal anode and an electrolyte, achieving good
electrochemical stability and high Coulombic efficiency for
metallic anodes.
2. Description of the Related Art
The concept of lithium ion batteries (LiBs) was first established
in 1962 and immediately lithium ion secondary batteries were
proposed by M. S. Whittingham from Exxon, leading to the invention
of Li--TiS.sub.2 batteries. However, the company failed to
commercialize battery systems including lithium metal and TiS.sub.2
as an anode and a cathode, respectively, because the lithium metal
(LiM) anode lacks in safety and the air/water sensitive TiS.sub.2
cathode incurs a high production cost.
These problems were solved by using graphite capable of reversible
intercalation and deintercalation of lithium as an anode and a
lithium transition metal oxide (developed by J. O Besenhard) as a
cathode, leading to the commercialization of current LiBs. The
first commercial LiB was released by Sony and Asahi Kasei in 1991
and brought about a milestone in successful market expansion of
portable electronic devices. Since then, the use of LiBs has
increased exponentially and met the demand for electrical energy
directly connected to consistent innovation of ordinary electronic
devices such as cell phones, music players, speakers, drones,
automobiles, and microsensors. Many researchers and scientists have
investigated and studied new advanced energy materials, chemistry,
and physics for stationary/mobile energy storage systems that meet
the increasing demand for energy.
The recent development of commercially available LiBs has reached a
saturation point. For example, only gradual improvements in the
electrochemical performance of LiBs have been reported. Thus, along
with the increasing demand for energy, research and development
needs to be conducted on new energy materials with different
morphologies and compositions. Under these circumstances, secondary
batteries such as lithium-sulfur and lithium-air batteries
including LiM anodes and conversion-type cathodes have received
attention as next-generation batteries due to their high energy
densities. Sulfur- and carbon-based air cathodes have theoretical
energy densities of .about.2,600 Wh/kg and .about.11,400 Wh/kg,
respectively, which are almost 10 times higher than that of LiBs
(.about.360 Wh/kg for C/LiCo.sub.2O.sub.4). LiM as an anode
material has a theoretical energy density as high as .about.3,860
Wh/kg, a very low redox potential of -3.04 V vs. S.H.E, and a
density of 0.59 g/cm.sup.3. In contrast, graphite is an anode
material that has a theoretical energy density as low as .about.372
mAh/g and is rather high in redox potential and density. Thus, the
use of a lithium anode instead of a graphite anode can contribute
to a significant increase in energy density per weight of LiB. Upon
successful commercialization of lithium-sulfur and lithium-air
batteries in the future, LiM anodes and conversion-type cathodes
are expected to meet the demand for high energy density.
Despite such advantages, several tough challenges should be
addressed to commercialize batteries using LiM anodes. The most
important challenge is to ensure reversible deposition and
dissolution of lithium. High reactivity and non-uniform deposition
of lithium cause many problems such as thermal runaway, electrolyte
decomposition, and lithium loss. Non-uniform deposition of lithium
during charge leads to the growth of dendrites that penetrate
separators, resulting in short-circuit. This short-circuit
generates much heat and sparks, causing serious safety problems
giving rise to ignition of flammable electrolytes. Other problems
of the LiM batteries are side reactions with electrolytes and
unstable Coulombic efficiency that cause the batteries to have low
capacity and poor life characteristics. This instability is caused
by continuous reactions between LiM and electrolytes to destroy and
form SEIs during continuous charge/discharge cycling. Such
undesired processes bring about continuous degradation of
electrolytes, and as a result, electrochemically inactive species
are formed in the batteries, deteriorating the performance of the
batteries. Therefore, there is a need to provide stable deposition
positions that form stable SEIs and protect the surface of active
lithium for stable deposition and dissolution of lithium. In this
scenario, the formation and growth of lithium dendrites can be
effectively suppressed. For this purpose, there have been made many
attempts. First, Cui and co-workers at Stanford University
artificially synthesized an interconnected hollow carbon sphere
film (200-300 nm thick) on the surface of lithium metal to isolate
the LiM from an electrolyte. The electrochemically and mechanically
stable artificial SEI layer, also called "hard-film", can suppress
the formation of lithium dendrites. Further, Archer and co-workers
at Cornell University proposed a dendrite-free lithium anode in
which LiF-coated Li reduces the growth of lithium dendrites and
forms a stable SEI. Many other effective chemical additives and
flexible SEI films have been proposed. However, processes for
producing protective films in an economical, easy, and more
effective manner still need to be developed to make LiM
commercially available as an anode material.
The present inventor has found that when a solid electrolyte
interphase of an anode for a secondary battery including
amino-functionalized carbon structures is formed on an anode
material in the form of a foil to protect the anode material,
lithium can be rapidly diffused and stably deposited to inhibit the
formation of dendrites in the anode. The present inventor has also
found that the solid electrolyte interphase prevents side reactions
between a lithium metal electrode and an electrolyte in a lithium
metal secondary battery using the anode, achieving good stability
and high Coulombic efficiency of the lithium metal secondary
battery.
PRIOR ART DOCUMENTS
Patent Documents
Patent Document 1: Korean Patent Publication No.
10-2014-0112597
Patent Document 2: Korean Patent Publication No.
10-2014-0089450
SUMMARY OF THE INVENTION
The present invention has been made in view of the above problems,
and it is an object of the present invention to provide an
artificial solid electrolyte interphase of an anode for a secondary
battery including amino-functionalized carbon structures to protect
an underlying anode material in the form of a foil, an anode for a
lithium metal secondary battery in which the artificial solid
electrolyte interphase is used so that lithium can be rapidly
diffused and stably deposited to inhibit the formation of
dendrites, and a lithium metal secondary battery using the anode in
which the solid electrolyte interphase prevents side reactions
between a lithium metal and an electrolyte, achieving good
electrochemical stability and high Coulombic efficiency for the
metallic anode.
One aspect of the present invention provides the artificial solid
electrolyte interphase (ASEI) of an anode for a secondary battery
including a multi-walled carbon nanotube thin film or a reduced
graphene oxide (rGO) thin film to protect the anode material.
A further aspect of the present invention provides an anode for a
secondary battery including an anode material in the form of a foil
and the artificial solid electrolyte interphase formed on the anode
material to protect the anode material wherein the artificial solid
electrolyte interphase contains multi-walled carbon nanotubes or
rGO.
Another aspect of the present invention provides a secondary
battery including the metallic anode.
Another aspect of the present invention provides an electric device
including the anode wherein the electric device is selected from
electric vehicles, hybrid electric vehicles, plug-in hybrid
electric vehicles, and energy storage systems.
Yet another aspect of the present invention provides a method for
producing an anode including (b) forming a
polyethyleneimine-attached amino-functionalized multi-walled carbon
nanotube (PEI MWCNT) thin film or a polyethyleneimine-bound
amino-functionalized rGO (PEIrGO) thin film and (c) transferring
the PEI MWCNT or PEIrGO thin film to the surface of the anode
material in the form of a foil.
The formation of the artificial solid electrolyte interphase
including amino-functionalized carbon structures on the anode
material in the form of the foil enables rapid diffusion and stable
deposition of lithium to suppress the formation of dendrites. In
addition, the artificial solid electrolyte interphase prevents side
reactions between the lithium metal anode and the electrolyte,
achieving good electrochemical stability and high Coulombic
efficiency for metallic anodes.
BRIEF DESCRIPTION OF THE DRAWINGS
These and/or other aspects and advantages of the invention will
become apparent and more readily appreciated from the following
description of the embodiments, taken in conjunction with the
accompanying drawings of which:
FIG. 1A is a schematic diagram of an anode produced in Example 1 in
which a polyethyleneimine-attached amino-functionalized
multi-walled carbon nanotube (PEI MWCNT) thin film was formed on a
lithium metal electrode;
FIG. 1B is a schematic diagram of an anode produced in Example 2 in
which a polyethyleneimine-bound amino-functionalized reduced
graphene oxide (PEIrGO) thin film was formed on a lithium metal
electrode;
FIG. 2A shows scanning electron microscopy (SEM) images of (a)
multi-walled carbon nanotubes (MWCNTs) used in Example 1 and (b)
PEI MWCNTs prepared in Example 1;
FIG. 2B is a scanning electron microscopy (SEM) image showing the
surface profile of a lithium metal electrode (PEIrGO Li) prepared
in Example 2;
FIG. 3 shows X-ray photoelectron spectra (XPS) of reduced graphene
oxide (rGO) synthesized in Example 2;
FIG. 4 shows X-ray photoelectron spectra (XPS) of
polyethyleneimine-bound amino-functionalized rGO (PEIrGO)
synthesized in Example 2;
FIG. 5A shows scanning electron microscopy (SEM) images showing the
surface morphologies of (a) pristine lithium metal of Comparative
Example 1 ("Pristine Li"), (b) an electrode in which a thin film of
MWCNTs used in Example 1 was formed on lithium metal ("MWCNT Li"),
and (c) an electrode of Example 1 in which a PEI MWCNT thin film
was formed on lithium metal ("PEI MWCNT Li");
FIG. 5B shows scanning electron microscopy (SEM) images showing the
surface morphologies of (a) pristine lithium metal of Comparative
Example 1 ("Pristine Li") and (b) a lithium metal electrode (PEIrGO
Li) produced in Example 2 after electrochemical cycles, and (c) a
higher magnification image of (b);
FIG. 6 shows a scanning electron microscopy (SEM) image and EDXS
elemental mapping images of PEI MWCNTs prepared in Example 1;
FIG. 7A shows a change in the weight of PEI by thermogravimetric
analysis (TGA) of PEI MWCNTs prepared in Example 1;
FIG. 7B shows a change in the weight of PEI by thermogravimetric
analysis (TGA) of polyethyleneimine-bound rGO (PEIrGO) prepared in
Example 2;
FIG. 8 is a scanning electron microscopy (SEM) image of MWCNTs
(diameter: 9.5 nm, length: 1.5 .mu.m) used in Comparative Example
2;
FIG. 9 shows the Coulombic efficiency of a lithium metal secondary
battery using a copper foil coated with PEI MWCNTs prepared in
Example 1 as a cathode and a lithium foil as an anode for lithium
deposition and dissolution reactions when charged/discharged with a
current density of 1 mA/cm.sup.2 and a capacity of 1
mAh/cm.sup.2;
FIG. 10 shows cycle characteristics and charge/discharge
efficiencies of a lithium metal secondary battery using a pristine
lithium metal electrode of Comparative Example 1 ("Pristine Li") as
an anode, a lithium metal secondary battery using an anode in which
a thin film of MWCNTs used in Example 1 was formed on lithium metal
("MWCNT Li"), a lithium metal secondary battery using an anode in
which a PEI MWCNT thin film prepared in Example 1 was formed on
lithium metal ("PEI MWCNT Li"), and a lithium metal secondary
battery using an anode in which a thin and small MWCNT thin film
prepared in Comparative Example 2 was formed ("Thin & Small
MWCNT");
FIG. 11 shows voltage profiles of a lithium metal secondary battery
using a pristine lithium metal electrode of Comparative Example 1
("Pristine Li") as an anode and a lithium metal secondary battery
using an anode in which a thin film of MWCNTs used in Example 1 was
formed on lithium metal ("MWCNT Li") at the 30th and 90th
cycles;
FIG. 12 shows cycle characteristics and charge/discharge
efficiencies of a lithium metal secondary battery using an NCM
electrode plate as a cathode and a pristine lithium metal electrode
of Comparative Example 1 ("Pristine Li") as an anode and a lithium
metal secondary battery using an NCM electrode plate as a cathode
and a lithium metal electrode (PEIrGO Li) as an anode; and
FIG. 13 shows voltage profiles of a lithium metal secondary battery
using a pristine lithium metal electrode of Comparative Example 1
("Pristine Li") as an anode and a lithium metal secondary battery
using a lithium metal electrode (PEIrGO Li) as an anode at the 30th
and 90th cycles.
DETAILED DESCRIPTION OF THE INVENTION
Several aspects and various embodiments of the present invention
will now be described in more detail.
One aspect of the present invention is directed to the artificial
solid electrolyte interphase (ASEI) of an anode for a secondary
battery including multi-walled carbon nanotube thin films or a
reduced graphene oxide (rGO) thin films to protect the metallic
anode material.
During electrochemical cycling of a lithium metal secondary
battery, highly active and flexible lithium metal (LiM) tends to
induce dendritic Li electrodeposits on the LiM anode during
charging due to formations of heterogeneous interphases at the
anode/electrolyte interface caused by uncontrolled lithium
migrations. Once the rough electrodeposits of the lithium formed on
the surface of the lithium metal, lithium dendrites penetrate a
separator to cause an internal short circuit. This short circuit
generates heat, causing the battery to explode. Particularly,
high-energy density lithium metal anode used in secondary batteries
have 10 folds higher energy densities than graphite anodes in
conventional lithium ion batteries. Accordingly, one of the most
crucial requirements for the commercialization of lithium metal
secondary batteries is to develop technologies for improving
battery safety while minimizing the danger of battery explosion.
Further, repeated charge/discharge cycles lead to an increase in
surface area, causing degradation of electrolytes. The continuous
destruction and reformation of SEI layers brings about loss of
lithium (i.e. low Coulombic efficiency).
The present invention is aimed at solving the aforementioned
problems. Specifically, the artificial solid electrolyte interphase
of the anode for the secondary battery including the multi-walled
carbon nanotube thin film or a reduced graphene oxide thin film is
formed on an anode material in the form of a foil to protect the
anode material. The artificial solid electrolyte interphase
suppresses the formation of lithium dendrites such that the lithium
metal as the anode is physicochemically stabilized and the
occurrence of an internal short circuit is prevented.
According to one embodiment, the multi-walled carbon nanotubes may
have a diameter of 50 to 300 nm, preferably 80 to 200 nm, more
preferably 110 to 170 nm and a length of 3 to 50 .mu.m, preferably
4 to 30 .mu.m, more preferably 5 to 90 .mu.m.
In the Examples section that follows, it was found that when the
multi-walled carbon nanotubes are less than 50 nm in diameter or 3
.mu.m in length, the electrochemical properties of an anode using
the multi-walled carbon nanotubes deteriorated. Further, when the
multi-walled carbon nanotubes were more than 300 nm in diameter or
50 .mu.m in length, the electrochemical properties of an anode
using the multi-walled carbon nanotubes deteriorated.
According to a further embodiment, the multi-walled carbon
nanotubes or rGO may be an amino-functionalized one.
According to another embodiment, the amino-functionalized
multi-walled carbon nanotubes or amino-functionalized rGO may be
prepared by binding the multi-walled carbon nanotubes or rGO with
polyethyleneimine.
Particularly, the amine groups present in the polyethyleneimine
attached to the multi-walled carbon nanotubes attract lithium due
to their high binding energy of lithium and allow the long and
intricately interconnected fibrous carbon nanotubes having a
3-dimensional structure to assist in stably plating/dissolving the
attracted lithium. The amine groups present in the
polyethyleneimine bound to the rGO attract lithium due to their
high binding energy of lithium and the two-dimensional structures
of the graphene are stacked to form a three-dimensional network.
The high conductivity of the three-dimensional network allows
lithium to migrate easily, ensuring stable plating/dissolution of
lithium in the lithium electrode. Therefore, the
amino-functionalized multi-walled carbon nanotubes or
amino-functionalized reduced graphene oxide suppresses the
formation of lithium dendrites and enhances the physicochemical
stability at a lithium/electrolyte interface. The formation of the
functional artificial solid electrolyte interphase ensures
continuous plating and dissolution of lithium in the lithium
electrode for a prolonged period of time and achieves good
electrochemical stability and high Coulombic efficiency.
According to another embodiment, the polyethyleneimine may be
present in an amount ranging from 0.1 to 10% by weight, based on
the total weight of the amino-functionalized multi-walled carbon
nanotubes, and the polyethyleneimine may be present in an amount
ranging from 1 to 50% by weight, based on the total weight of the
amino-functionalized rGO.
Outside each range, satisfactory electrochemical properties of an
anode cannot be expected.
According to another embodiment, the solid electrolyte interphase
may further include a multi-walled carbon nanotube thin film
stacked on the rGO thin film.
According to another embodiment, the multi-walled carbon nanotubes
may be polyethyleneimine-attached amino-functionalized ones (PEI
MWCNTs).
The PEI MWCNTs form a buffer layer through which the rGO thin film
or PEIrGO thin film can be uniformly transferred to the surface of
the lithium metal. The buffer layer can assist in easily peeling
off a filter paper to which the rGO or PEIrGO adheres well when the
rGO or PEIrGO is transferred to the lithium.
Particularly, although not explicitly described in the Examples
section that follows, the artificial solid electrolyte interphases
of anodes for secondary batteries including multi-walled carbon
nanotubes to protect anode materials were formed by varying the
diameter and length of the multi-walled carbon nanotubes and
conditions for amino functionalization of the multi-walled carbon
nanotubes, the artificial solid electrolyte interphases were
applied to anodes for lithium metal secondary batteries, lithium
metal secondary batteries fabricated using the anodes were operated
at high temperature for 500 hours, and then the cross-sections of
the anodes were observed and losses of the multi-walled carbon
nanotubes were investigated by scanning electron microscopy
(SEM).
As a result, when the following conditions were met, no voids were
formed at the interfaces between the lithium metal and the
artificial solid electrolyte interphases and no losses of the
multi-walled carbon nanotubes coated on the lithium metal were
found even after the lithium metal secondary batteries were
operated at high temperature for 500 hours, unlike when other
conditions and other numerical ranges were employed: (i) the
multi-walled carbon nanotubes had a diameter of 50 to 300 nm; (ii)
the multi-walled carbon nanotubes had a length of 3 to 50 .mu.m;
(iii) the multi-walled carbon nanotubes were amino-functionalized
by binding with polyethyleneimine; and (iv) the polyethyleneimine
was present in an amount of 0.1 to 10% by weight, based on the
total weight of the amino-functionalized multi-walled carbon
nanotubes.
When any one of the above conditions was not met, voids were formed
in many portions of the interfaces between the lithium metal and
the artificial solid electrolyte interphases and considerable
losses of the multi-walled carbon nanotubes coated on the lithium
metal electrodes were found after the lithium metal secondary
batteries were operated at high temperature for 500 hours.
Although not explicitly described in the Examples section that
follows, the artificial solid electrolyte interphases of anodes for
secondary batteries including reduced graphene oxide thin films to
protect anode materials were formed by varying the conditions for
amino functionalization and with or without additional thin films
stacked on the reduced graphene oxide thin films, the artificial
solid electrolyte interphases were applied to anodes for lithium
metal secondary batteries, lithium metal secondary batteries
fabricated using the anodes were operated at high temperature for
500 hours, and then the cross-sections of the anodes were observed
and losses of the reduced graphene oxide thin films were
investigated by scanning electron microscopy (SEM).
As a result, when the following conditions were met, no voids were
formed at the interfaces between the lithium metal and the
artificial solid electrolyte interphases and no losses of the
reduced graphene oxide thin films coated on the lithium metal were
found even after the lithium metal secondary batteries were
operated at high temperature for 500 hours, unlike when other
conditions and other numerical ranges were employed: (i) the rGO
was amino-functionalized by binding with polyethyleneimine; (ii)
the polyethyleneimine was present in an amount of 1 to 50% by
weight, based on the total weight of the amino-functionalized rGO;
(iii) the artificial solid electrolyte interphases further included
multi-walled carbon nanotube thin films stacked on the rGO thin
films; and (iv) the multi-walled carbon nanotubes were
amino-functionalized by binding with polyethyleneimine.
When any one of the above conditions was not met, voids were formed
in many portions of the interfaces between the lithium metal and
the artificial solid electrolyte interphases and considerable
losses of the reduced graphene oxide thin films coated on the
lithium metal electrodes were found after the lithium metal
secondary batteries were operated at high temperature for 500
hours.
A further aspect of the present invention is directed to an anode
including an anode material in the form of a foil and a solid
electrolyte interphase formed on the anode material to protect the
anode material wherein the artificial solid electrolyte interphase
contains multi-walled carbon nanotubes or rGO.
Only lithium is illustrated as the anode material in the drawings.
However, it should be understood that various metals such as
magnesium, sodium, potassium, and aluminum may be used as anode
materials of secondary batteries. Preferably, the anode material
foil is a lithium foil.
Another aspect of the present invention is directed to a secondary
battery including the anode.
The secondary battery may be selected from lithium metal secondary
batteries, lithium-sulfur batteries, lithium-air batteries, lithium
ion batteries, magnesium ion batteries, sodium ion batteries,
potassium ion batteries, and aluminum ion batteries. Preferably,
the secondary battery is a lithium metal secondary battery.
According to a further embodiment, a cathode of the secondary
battery may include at least one compound selected from lithium
cobalt oxides, lithium manganese oxides, lithium nickel cobalt
aluminum oxides, lithium nickel manganese cobalt oxides, lithium
iron phosphate oxide, and sulfur compounds or may be a porous air
electrode.
Another aspect of the present invention is directed to an electric
device including the anode wherein the electric device is selected
from electric vehicles, hybrid electric vehicles, plug-in hybrid
electric vehicles, and energy storage systems.
Yet another aspect of the present invention is directed to a method
for producing an anode including (b) forming a
polyethyleneimine-attached amino-functionalized multi-walled carbon
nanotube (PEI MWCNT) thin film or a polyethyleneimine-bound
amino-functionalized rGO (PEIrGO) thin film and (c) transferring
the PEI MWCNT or PEIrGO thin film to the surface of an anode
material in the form of a foil.
According to one embodiment, in step (b), a suspension of PEI
MWCNTs or PEIrGO may be coated on a substrate to form a PEI MWCNT
thin film or a PEIrGO thin film by a technique selected from vacuum
filtration, Langmuir-Blodgett scooping (LBS), tape casting,
electrospinning, spin coating, spray coating, and flat rolling.
There is no restriction on the technique for forming the PEI MWCNT
thin film. Preferably, the thin film is formed on a filter paper as
the substrate by vacuum filtration.
Langmuir-Blodgett scooping (LBS) refers to a process for forming a
thin film based on self-assembly of film-forming particles,
diffusion of a water-immiscible solvent, and the surface tension
gradient known as the "Marangoni effect". When a suspension of
film-forming particles in ethanol or isopropanol as a suspension
medium is injected into the surface of water, the suspension is
rapidly diffused on the water surface while interacting with the
water surface, lowering the surface tension of the water. As a
result, the particles slip and self-assemble. This self-assembly
leads to the formation of a uniform film on the water surface
during injection of the suspension.
According to a further embodiment, the method may further include
drying the thin film obtained by vacuum filtration at 30 to
150.degree. C. for 12 to 36 hours.
According to a further embodiment, in step (b), the PEIrGO thin
film is formed on a polyethyleneimine-bound amino-functionalized
multi-walled carbon nanotube (PEI MWCNT) thin film.
According to another embodiment, step (b) may include (A) applying
a suspension containing PEI MWCNTs to a filter paper, followed by
vacuum filtration to form a PEI MWCNT thin film on the filter paper
and (B) applying a suspension containing PEIrGO to the filter paper
on which the PEI MWCNT thin film is formed, followed by vacuum
filtration to form a PEIrGO thin film on the PEI MWCNT thin
film.
According to another embodiment, the method may further include
drying the PEIrGO thin film formed on the PEI MWCNT thin film by
vacuum filtration at 30 to 150.degree. C. for 12 to 36 hours.
According to another embodiment, the method may further include,
prior to step (b), (a) mixing multi-walled carbon nanotubes with
polyethyleneimine in a weight ratio of 1:0.1-5, preferably 1:0.5-3,
more preferably 1:1-2 and allowing the mixture to react at 50 to
150.degree. C., preferably 60 to 130.degree. C., more preferably 80
to 100.degree. C. for 12 to 36 hours, preferably 18 to 30 hours,
more preferably 22 to 26 hours to obtain polyethyleneimine-attached
amino-functionalized multi-walled carbon nanotubes.
Particularly, in the Examples section that follows, it was found
that when the weight ratio and the reaction temperature and time
were within the preferred ranges defined above, the largest
possible amount of the polyethyleneimine was attached to the
multi-walled carbon nanotubes.
According to another embodiment, the method may further include,
prior to step (b), ((a)-I) mixing rGO with polyethyleneimine in a
weight ratio of 1:0.1-5, preferably 1:0.5-3, more preferably 1:1-2
and allowing the mixture to react at 50 to 150.degree. C.,
preferably 60 to 130.degree. C., more preferably 80 to 100.degree.
C. for 12 to 36 hours, preferably 18 to 30 hours, more preferably
22 to 26 hours to obtain polyethyleneimine-bound
amino-functionalized rGO (PEIrGO) and ((a)-II) mixing multi-walled
carbon nanotubes with polyethyleneimine in a weight ratio of
1:0.1-5, preferably 1:0.5-3, more preferably 1:1-2 and allowing the
mixture to react at 50 to 150.degree. C., preferably 60 to
130.degree. C., more preferably 80 to 100.degree. C. for 12 to 36
hours, preferably 18 to 30 hours, more preferably 22 to 26 hours to
obtain polyethyleneimine-attached amino-functionalized multi-walled
carbon nanotubes (PEI MWCNTs).
Particularly, in the Examples section that follows, it was found
that when the weight ratio and the reaction temperature and time
were within the preferred ranges defined above, the largest
possible amount of the polyethyleneimine was attached to the rGO
and multi-walled carbon nanotubes.
According to another embodiment, step (c) may be carried out by
transferring the polyethyleneimine-attached amino-functionalized
multi-walled carbon nanotube thin films to the surface of lithium
metal. The polyethyleneimine-attached amino-functionalized
multi-walled carbon nanotube thin film, the PEIrGO thin film or the
PEIrGO thin film formed on the PEI MWCNT thin film to the surface
of lithium metal. The thin film may be provided in plurality.
According to another embodiment, step (c) may be carried out by
rolling.
According to another embodiment, in step (c), the lithium metal and
the filter paper may be pressed against each other such that the
lithium metal is close to the polyethyleneimine-attached
amino-functionalized multi-walled carbon nanotube thin film, the
PEIrGO thin film or the PEIrGO thin film formed on the PEI MWCNT
thin film.
According to another embodiment, the lithium metal and the filter
paper may be pressed against each other by sandwiching the lithium
metal and the filter paper close to each other between a pair of
protective films and passing the sandwich structure through a
rolling mill.
According to another embodiment, roll cylinders of the rolling mill
may be arranged at an interval corresponding to 50 to 90% of the
total thickness of all layers inserted therebetween and the rolling
speed of the rolling mill may be maintained at 0.05 to 0.2
cm/sec.
According to another embodiment, step (c) may further include
removing the filter paper from the lithium metal to which the
polyethyleneimine-attached amino-functionalized multi-walled carbon
nanotube thin film, the PEIrGO thin film or the PEIrGO thin film
formed on the PEI MWCNT thin film is transferred.
According to another embodiment, step (c) may be carried out in a
dry atmosphere at a relative humidity of 0% to 1%. If the relative
humidity exceeds the upper limit defined above, the lithium metal
may be oxidized, impairing the electrochemical properties of the
anode.
According to another embodiment, step (c) may be carried out in an
atmosphere of at least one inert gas selected from argon, nitrogen,
helium, and neon to prevent the oxidation and side reactions of the
lithium metal.
According to another embodiment, the filter paper may be
Celgard.RTM. 2500 or a polyethylene, polypropylene or polyolefin
separator. The filter paper is not limited but is preferably
Celgard.RTM. 2500.
According to another embodiment, the protective films may be
polyester or polycarbonate films. The protective films are not
limited but are preferably polyester films.
Particularly, although not explicitly described in the Examples
section that follows, anodes for lithium metal secondary batteries
in which artificial solid electrolyte interphases including
multi-walled carbon nanotubes are formed to protect the anode
materials were produced by varying the conditions defined in steps
(b) and (c), lithium metal secondary batteries fabricated using the
anodes were operated at a high temperature of 800.degree. C. or
above, and the morphologies of the anodes were observed by scanning
electron microscopy (SEM).
As a result, when the following conditions were met, no aggregation
of the polyethyleneimine-attached amino-functionalized multi-walled
carbon nanotube particles coated on the anode material foils was
observed even after the lithium metal secondary batteries were
operated at high temperature for 800 hours, demonstrating excellent
thermal stability of the secondary batteries, unlike when other
conditions and other numerical ranges were employed: (i) the thin
film obtained by vacuum filtration was dried at 30 to 150.degree.
C. for 12 to 36 hours; (ii) the polyethyleneimine-attached
amino-functionalized multi-walled carbon nanotubes were prepared by
mixing multi-walled carbon nanotubes with polyethyleneimine in a
weight ratio of 1:1-2 and allowing the mixture to react at 80 to
100.degree. C. for 22 to 26 hours; (iii) a lithium foil was used as
the anode material foil; (iv) step (c) was carried out by rolling,
(v) roll cylinders of the rolling mill were arranged at an interval
corresponding to 50 to 90% of the total thickness of all layers
inserted therebetween; (vi) the rolling speed of the rolling mill
were maintained at 0.05 to 0.2 cm/sec; (vii) step (c) was carried
out in a dry atmosphere at a relative humidity of 0% to 1%; (viii)
Celgard.RTM. 2500 was used as the filter paper; and (ix) polyester
films was used as the protective films.
When any one of the above conditions was not met, considerable
aggregation of the polyethyleneimine-attached amino-functionalized
multi-walled carbon nanotube particles coated on the lithium metal
was observed after the lithium metal secondary batteries were
operated at high temperature for 800 hours.
Particularly, although not explicitly described in the Examples
section that follows, anodes for lithium metal secondary batteries
containing artificial solid electrolyte interphases in which PEIrGO
thin films were formed on PEI MWCNT thin films to protect the anode
materials were produced by varying the conditions defined in steps
(b) and (c), lithium metal secondary batteries fabricated using the
anodes were operated at a high temperature of 800.degree. C. or
above, and the morphologies of the anodes were observed by scanning
electron microscopy (SEM).
As a result, when the following conditions were met, no aggregation
of the polyethyleneimine-bound amino-functionalized reduced
graphene oxide (PEIrGO) particles coated on the anode material
foils was observed even after the lithium metal secondary batteries
were operated at high temperature for 800 hours, demonstrating
excellent thermal stability of the secondary batteries, unlike when
other conditions and other numerical ranges were employed: (i) in
step (b), (A) a suspension containing PEI MWCNTs was applied to a
filter paper, followed by vacuum filtration to form a PEI MWCNT
thin film on the filter paper and (B) a suspension containing
PEIrGO was applied to the filter paper on which the PEI MWCNT thin
film was formed, followed by vacuum filtration to form a PEIrGO
thin film on the PEI MWCNT thin film; (ii) the PEIrGO thin film
formed on the PEI MWCNT thin film by vacuum filtration was dried at
30 to 150.degree. C. for 12 to 36 hours; (iii) the
polyethyleneimine-bound amino-functionalized rGO was prepared by
mixing rGO with polyethyleneimine in a weight ratio of 1:1-2 and
allowing the mixture to react at 80 to 100.degree. C. for 22 to 26
hours; (iv) the polyethyleneimine-bound amino-functionalized
multi-walled carbon nanotubes were prepared by mixing multi-walled
carbon nanotubes with polyethyleneimine in a weight ratio of 1:1-2
and allowing the mixture to react at 80 to 100.degree. C. for 22 to
26 hours; (v) a lithium foil was used as the anode material foil;
(vi) step (c) was carried out by rolling, (vii) roll cylinders of
the rolling mill were arranged at an interval corresponding to 50
to 90% of the total thickness of all layers inserted therebetween;
(viii) the rolling speed of the rolling mill were maintained at
0.05 to 0.2 cm/sec; (ix) step (c) was carried out in a dry
atmosphere at a relative humidity of 0% to 1%; (x) a polyolefin
separator was used as the filter paper; and (xi) polyester films
was used as the protective films.
When any one of the above conditions was not met, considerable
aggregation of the PEIrGO particles coated on the lithium metal was
observed after the lithium metal secondary batteries were operated
at high temperature for 800 hours.
The present invention will be explained in detail with reference
with the following examples in conjunction with the accompanying
drawings.
Example 1: Production of Lithium Metal Electrode on which
Polyethyleneimine-Attached Amino-Functionalized Multi-Walled Carbon
Nanotube Thin Film was Formed
(1) Preparation of Polyethyleneimine-Attached Amino-Functionalized
Multi-Walled Carbon Nanotube (PEI MWCNT) Thin Film
1 g of commercial MWCNTs (diameter: 110-170 nm, length: 5-9 .mu.m)
were allowed to react with 1.5 g of polyethyleneimine (average Mw:
.about.60,000 (LS), average Mn: .about.750,000 (GPC), branched) in
deionized water with stirring at 90.degree. C. for 24 h. The
reaction mixture was cooled to room temperature, centrifuged 10
times, and washed to obtain polyethyleneimine-bound
amino-functionalized multi-walled carbon nanotubes ("PEI
MWCNTs").
3 mg of the PEI MWCNT nanoparticles were dissolved in 30 mL of
ethanol to prepare a suspension. Thereafter, the suspension was
formed into a PEI MWCNT thin film by vacuum filtration using a
separator (Celgard.RTM. 2500) filter paper. The thin film was dried
in an oven at 60.degree. C. for 1 day.
(2) Transfer of the Thin Film to Lithium Metal Electrode
The PEI MWCNT thin film formed on the Celgard.RTM. 2500 filter
paper was transferred to the surface of lithium metal using a
rolling mill. Specifically, under dry environmental conditions,
lithium metal and the PEI MWCNT thin film were sandwiched between
Mylar films (polyester films) and the sandwich structure was
uniformly pressurized in a rolling mill. The interval between rolls
of the rolling mill was adjusted to 0.1 mm (corresponding to 70% of
the total thickness of all layers inserted between the rolls) and
the rolling speed of the rolling mill was maintained at 0.1 cm/sec.
Thereafter, the Mylar films were removed, the filter paper attached
to the lithium metal was peeled off, and then the electrochemical
properties of the PEI MWCNT thin film-transferred lithium metal
were measured. The entire procedure was carried out in a dry
atmosphere at a relative humidity of 0-1% and an inert gas
atmosphere.
Example 2: Production of Lithium Metal Electrode on which
Polyethyleneimine-Bound Amino-Functionalized Reduced Graphene Oxide
(rGO) was Formed
(1) Material Synthesis
First, graphite flakes were dispersed in concentrated sulfuric acid
and cooled to 0.degree. C. Then, 5 g of a potassium permanganate
solution was added dropwise to the dispersion while preventing the
temperature from exceeding 10.degree. C., and distilled water and
aqueous hydrogen peroxide were added thereto. The resulting
solution was filtered several times with deionized water to obtain
graphene oxide. The graphene oxide powder was treated with hydrogen
gas in a tube furnace at 800.degree. C. for 120 min while feeding
the hydrogen gas at a flow rate of 100 sccm and was allowed to cool
to room temperature to obtain reduced graphene oxide. The reduced
graphene oxide was sufficiently dispersed in water to attach PEI
thereto. To this end, 400 mg of the reduced graphene oxide was
added to 500 ml of distilled water and was then sufficiently
dispersed by sonication for 30 min The dispersion was added with
0.5 g of polyethyleneimine (average Mw .about.60,000 (LS), average
Mn .about.750,000 (GPC), branched). The resulting solution was kept
in a thermostatic oil bath at 90.degree. C. for 24 h with stirring,
cooled to room temperature, and washed ten times with deionized
water by centrifugation to synthesize polyethyleneimine-bound
amino-functionalized rGO.
Next, 1 g of MWCNTs (diameter: 110-170 nm, length: 5-9 .mu.m) were
allowed to react with 1 g of polyethyleneimine (average Mw
.about.60,000 (LS), average Mn .about.750,000 (GPC), branched) in
deionized water at 90.degree. C. for 24 h. The reaction mixture was
cooled to room temperature and washed 10 times by centrifugation to
synthesize polyethyleneimine-attached amino-functionalized
multi-walled carbon nanotubes (PEI MWCNTs).
(2) Thin Film Formation
1 g of the PEI MWCNT nanoparticles were dissolved in 30 mL of
ethanol to prepare a suspension. Thereafter, the suspension was
applied to a separator (Celgard.RTM. 2500, Nafion-coated
polypropylene separator) filter paper, followed by vacuum
filtration to form a PEI MWCNT thin film on the filter paper.
Subsequently, 2 mg of PEIrGO nanoparticles were dissolved in 30 mL
of ethanol to prepare a suspension. Thereafter, the suspension was
applied to the PEI MWCNT thin film formed on the filter paper,
followed by vacuum filtration to form a PEIrGO thin film on the PEI
MWCNT thin film. The resulting thin film was dried in an oven at
60.degree. C. for 1 day.
(3) Transfer of the Thin Film to Lithium Metal Electrode
The PEIrGO thin film was transferred from the PEI MWCNT thin film
formed on the Celgard.RTM. 2500 filter paper to the surface of
lithium metal using a rolling mill. Specifically, under dry
environmental conditions, lithium metal and the PEIrGO thin film
were sandwiched between Mylar films (polyester films) and the
sandwich structure was uniformly pressurized in a rolling mill. The
interval between rolls of the rolling mill was adjusted to 0.1 mm
(corresponding to 70% of the total thickness of all layers inserted
between the rolls) and the rolling speed of the rolling mill was
maintained at 0.1 cm/sec. Thereafter, the Mylar films were removed,
the filter paper attached to the lithium metal was peeled off, and
then the electrochemical properties of the lithium metal to which
the PEIrGO thin film was transferred from the PEI MWCNT thin film
were measured. The entire procedure was carried out in a dry
atmosphere at a relative humidity of 0-1% and an inert gas
atmosphere.
Comparative Example 1: Pristine Lithium Metal Electrode
A pristine lithium metal electrode ("Pristine Li") without a PEI
MWCNT thin film was prepared.
Comparative Example 2: Lithium Metal Electrode Using Thin and Small
MWCNTs
3 mg of MWCNT nanoparticles (diameter: 9.5 nm, length: 1.5 .mu.m)
were dissolved in 30 mL of ethanol to prepare a suspension.
Thereafter, the suspension was formed into a thin film by vacuum
filtration using a separator (Celgard.RTM. 2500) filter paper. The
thin film was dried in an oven at 60.degree. C. for 1 day.
Thereafter, a lithium metal electrode was produced in the same
manner as in (2) of Example 1.
FIG. 1A is a schematic diagram of the anode produced in Example 1
in which the polyethyleneimine-attached amino-functionalized
multi-walled carbon nanotube (PEI MWCNT) thin film was formed on
the lithium metal electrode. FIG. 1B is a schematic diagram of the
anode produced in Example 2 in which the polyethyleneimine-bound
amino-functionalized reduced graphene oxide (PEIrGO) thin film was
formed on the lithium metal electrode.
As shown in FIGS. 1a and 1b, lithium was uniformly plated through
the artificial solid electrolyte interphase based on the
PEI-attached MWCNTs or rGO. The amine groups abundantly present in
the PEI are functional groups that attract lithium due to their
polarity. The amine groups attracting lithium greatly stabilize the
migration, plating, and dissolution of the lithium through the
highly conductive, long and intricate layered structure and
three-dimensional network of the MWCNTs or rGO.
FIG. 2A shows scanning electron microscopy (SEM) images of the
multi-walled carbon nanotubes (MWCNTs) used in Example 1 (a) and
the PEI MWCNTs prepared in Example 1 (b). FIG. 2B is a scanning
electron microscopy (SEM) image showing the surface profile of the
lithium metal electrode (PEIrGO Li) prepared in Example 2.
Referring to FIG. 2A, the structure of the MWCNTs was almost
completely conserved even after attachment of the PEI.
FIG. 2B shows the formation of the uppermost thin PEI MWCNT layer
and the presence of the underlying PEIrGO. The PEI MWCNTs form a
buffer layer through which the PEIrGO can be uniformly transferred
to the surface of the lithium metal. The buffer layer can assist in
easily peeling off the filter paper to which the PEIrGO adheres
well when the PEIrGO is transferred to the lithium.
FIG. 3 shows X-ray photoelectron spectra (XPS) of the reduced
graphene oxide (rGO) synthesized in Example 2.
Referring to FIG. 3, binding points for C and O of the rGO were
clearly observed as expected. Binding points for N were not
observed due to the absence of amine groups (i.e. N binding
points).
FIG. 4 shows X-ray photoelectron spectra (XPS) of the
polyethyleneimine-bound amino-functionalized rGO (PEIrGO)
synthesized in Example 2;
Referring to FIG. 4, binding points for C, O, and N of the PEIrGO
were clearly observed as expected. N binding points were also
clearly observed, indicating the attachment of the PEI to the
graphene.
FIG. 5A shows scanning electron microscopy (SEM) images showing the
surface morphologies of the pristine lithium metal of Comparative
Example 1 ("Pristine Li") (a), the electrode in which the MWCNT
thin film used in Example 1 was formed on lithium metal ("MWCNT
Li") (b), and the electrode of Example 1 in which the PEI MWCNT
thin film was formed on lithium metal ("PEI MWCNT Li") (c).
Referring to (a) of FIG. 5A, lithium dendrites were formed in the
anode using the pristine lithium owing to the instability of the
lithium surface. (b) of FIG. 5A reveals that the formation and
diffusion of dendrites was less observed in the lithium anode in
which the artificial solid electrolyte interphase based on the
MWCNTs having a three-dimensional structure was formed. (c) of FIG.
5A reveals that lithium was more smoothly plated in the lithium
anode including an artificial solid electrolyte interphase based on
the PEI-functionalized MWCNTs, resulting in more stable migration
and plating of the lithium than in the lithium anode based on the
MWCNTs.
FIG. 5B shows scanning electron microscopy (SEM) images showing the
surface morphologies of the pristine lithium metal of Comparative
Example 1 ("Pristine Li") (a) and the lithium metal electrode
(PEIrGO Li) produced in Example 2 after electrochemical cycles (b).
(c) of FIG. 5B is a higher magnification image of (b);
Referring to FIG. 5B, lithium dendrites were formed in the anode
using the pristine lithium (Pristine Li) (a) owing to the
instability of the lithium surface. In contrast, the formation and
diffusion of dendrites was less observed in the lithium anode in
which an artificial solid electrolyte interphase based on the
PEIrGO having a three-dimensional structure was formed (b). The
higher magnification image (c) shows smooth plating of lithium. In
conclusion, more stable migration, plating, and dissolution of
lithium was achieved in the electrode including the PEIrGO thin
film than in the pristine Li anode.
FIG. 6 shows a scanning electron microscopy (SEM) image and EDXS
elemental mapping images of the PEI MWCNTs prepared in Example
1.
Referring to FIG. 6, the PEI MWCNTs were primarily composed of C
and O and the presence of N resulting from the attachment of PEI
was confirmed.
FIG. 7A shows a change in the weight of PEI by thermogravimetric
analysis (TGA) of the PEI MWCNTs prepared in Example 1. FIG. 7B
shows a change in the weight of PEI by thermogravimetric analysis
(TGA) of the polyethyleneimine-bound rGO (PEIrGO) prepared in
Example 2.
FIG. 7A reveals that the content of PEI in the PEI MWCNTs was
.about.1 wt %. In contrast, the weight of the PEI-free MWCNTs
remained unchanged despite the change in temperature.
FIG. 7B reveals that the content of PEI in the PEIrGO was .about.25
wt %.
FIG. 8 is a scanning electron microscopy (SEM) image of the MWCNTs
(diameter: 9.5 nm, length: 1.5 .mu.m) used in Comparative Example
2.
An artificial solid electrolyte interphase based on the dense
MWCNTs shown in FIG. 8 will deteriorate the electrochemical
properties of a lithium metal battery due to the very small size of
voids through which lithium can migrate across the artificial solid
electrolyte interphase. That is, the presence of very small voids
leads to an increase in surface resistance with increasing number
of cycles. This explains better electrochemical properties of the
carpet-like MWCNT structure with large voids shown in FIG. 2 (see
FIG. 10).
FIG. 9 shows the Coulombic efficiency of a lithium metal secondary
battery using a copper foil coated with the PEI MWCNTs prepared in
Example 1 as a cathode and a lithium foil as an anode for lithium
deposition and dissolution reactions when charged/discharged with a
current density of 1 mA/cm.sup.2 and a capacity of 1
mAh/cm.sup.2.
Referring to FIG. 9, the Coulombic efficiency of a copper electrode
without PEI MWCNTs was rapidly reduced after the 30.sup.th cycle
whereas that of the copper electrode coated with the PEI MWCNTs was
maintained at 90% of its initial value even after the 200.sup.th
cycle.
FIG. 10 shows cycle characteristics and charge/discharge
efficiencies of a lithium metal secondary battery using an NCM
electrode plate as a cathode (see FIG. 12) and the pristine lithium
metal electrode of Comparative Example 1 ("Pristine Li") as an
anode, a lithium metal secondary battery using the anode in which
the MWCNT thin film used in Example 1 was formed on lithium metal
("MWCNT Li"), a lithium metal secondary battery using the anode in
which the PEI MWCNT thin film prepared in Example 1 was formed on
lithium metal ("PEI MWCNT Li"), and a lithium metal secondary
battery using the anode in which the thin and small MWCNT thin film
prepared in Comparative Example 2 was formed ("Thin & Small
MWCNT").
0.6 M LiTFSI, 0.4 M LiBOB, and 0.05 M LiPF.sub.6 were added to a
solution of EC and DMC (4:6 wt %) to prepare an electrolyte. Each
of the anodes and the electrolyte were used to construct a cell.
The cycle characteristics and charge/discharge efficiency of the
cell were measured at 1 C.
Referring to FIG. 10, the life of the cell using the pristine
lithium anode was only 100 cycles whereas the lives of the cells
using the lithium anode coated with the MWCNTs and the lithium
anode coated with the PEI MWCNTs were 170 cycles and >250
cycles, respectively. However, the cell using the anode coated with
the thin and small MWCNTs shown in FIG. 8 showed a shorter cycle
life than the cell using the pristine lithium anode. This result is
believed to be due to the very small size of voids through which
lithium can migrate across the SEI. That is, the presence of very
small voids leads to an increase in the surface resistance of the
anode, causing poor performance of the lithium metal secondary
battery.
FIG. 11 shows voltage profiles of a lithium metal secondary battery
using the pristine lithium metal electrode of Comparative Example 1
("Pristine Li") as an anode and a lithium metal secondary battery
using the anode in which the MWCNT thin film used in Example 1 was
formed on lithium metal ("MWCNT Li") at the 30.sup.th and 90.sup.th
cycles.
Referring to FIG. 11, the formation of dendrites in the pristine
lithium anode led to SEI build-up, resulting in higher overvoltages
at the 90.sup.th cycle than at the 30.sup.th cycle. In contrast,
the overvoltages of the lithium metal of the lithium anode coated
with the PEI MWCNTs were relatively well maintained. These results
are attributed to the formation of a stable SEI that suppresses the
growth of lithium dendrites, confirming that the surface resistance
of lithium remained substantially unchanged despite the increased
number of cycles.
FIG. 12 shows cycle characteristics and charge/discharge
efficiencies of the lithium metal secondary battery using an NCM
electrode plate as a cathode and the pristine lithium metal
electrode of Comparative Example 1 ("Pristine Li") as an anode and
a lithium metal secondary battery using an NCM electrode plate as a
cathode and the lithium metal electrode (PEIrGO Li) as an
anode.
Each of the lithium metal secondary batteries was fabricated by the
following procedure. First, a cathode plate was produced using
LiNiCoMnO.sub.2 (NCM) [8/1/1] as a three-component system. To this
end, the synthesized sample (92%), a conductive material (4%), and
a binder (4%) composed of polyvinylpyrrolidone (Mw .about.360,000)
(2%), polyethylene oxide (Mw .about.1,000,000) (1%), sodium
carboxymethyl cellulose (Mw .about.250,000) (1%) were mixed in
water to prepare a slurry. The slurry was coated on an aluminum
foil using a doctor blade, dried in an oven at 80.degree. C. for 1
day, and cut into a circular disc, which was used as a cathode.
Pristine Li or PEIrGO Li was used as an anode and Celgard.RTM. 2500
was used as a separator. A solution of 0.6 M LiTFSI, 0.4 M LiF, 0.4
M LiBOB, and 0.05 M LiPF.sub.6 in EC and DMC (4:6 wt %) was used as
an electrolyte. The cathode active material NCM was loaded at a
surface density of 18 mg/cm.sup.2. The cathode, anode, separator,
and electrolyte were assembled into a 2032 coin cell. The battery
was subjected to a charge/discharge test at 1 C using a Maccor
battery tester.
Referring to FIG. 12, the life of the cell using the pristine
lithium anode was 100 cycles, whereas the life of the cell using
the PEIrGO-coated lithium anode was >170 cycles.
FIG. 13 shows voltage profiles of a lithium metal secondary battery
using the pristine lithium metal electrode of Comparative Example 1
("Pristine Li") as an anode and a lithium metal secondary battery
using the lithium metal electrode (PEIrGO Li) as an anode at the
30.sup.th and 90.sup.th cycles.
Referring to FIG. 13, the formation of dendrites in the pristine
lithium anode led to SEI build-up, resulting in higher overvoltages
at the 90.sup.th cycle than at the 30.sup.th cycle. In contrast,
the overvoltages of the lithium metal anode coated with the PEIrGO
were relatively well maintained. These results are attributed to
the formation of a stable SEI that suppresses the growth of lithium
dendrites, confirming that the surface resistance of lithium
remained substantially unchanged despite the increased number of
cycles.
In conclusion, the formation of the polyethyleneimine-attached
amino-functionalized multi-walled carbon nanotube (PEI MWCNT) thin
film or the polyethyleneimine-bound amino-functionalized reduced
graphene oxide (PEIrGO) thin film on the lithium metal electrode in
the anode of the present invention enables rapid diffusion and
stable deposition of lithium to inhibit the formation of dendrites.
In addition, the presence of the PEI MWCNT thin film or the PEIrGO
thin film prevents side reactions between the lithium metal anode
and the electrolyte in the lithium metal secondary battery of the
present invention, achieving good electrochemical stability and
high Coulombic efficiency of the lithium metal secondary
battery.
* * * * *